Flash and JavaScript are required for this feature.
Download the video from Internet Archive.
Description
Professor Imperiali begins with a wrap-up of the previous lecture on enzymes, and then moves on to discussing carbohydrates.
Instructor: Barbara Imperiali
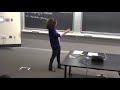
Lecture 5: Carbohydrates an...
[SOUND EFFECTS]
PROFESSOR: OK, I just want to just highlight your attention to things. Like every day, I get that MIT news feed. I get a couple of other news feeds. And I just thought this was really sort of a striking image and I think a great way to convey science and engineering is through sort of really eye catching imagery.
This is a synapse, which is where nerves contact to other nerves or to the neuromuscular junction in order to trigger activity. And this is a piece of research out of the Cima-- whoops, go back one-- whoops, go back one more-- between the Cima and Langer labs, where they've designed little tiny microchip probes.
They're about 10 microns big. They can be planted in the brain in different sites, hopefully non-invasively. And they can report on concentrations of this neurotransmitter, dopamine.
And the reason you might want to be able to do that is that there is a dopamine deficit in a lot of neurologic disorders. So you would like to understand what the deficits are and pinpoint points of the brain where there may be issues.
And in fact, they used it to track Parkinson's disease. Because Parkinson's disease, some of the therapeutic approaches involve deep brain stimulation. But you can't really tell if it's working unless you can measure something. And the thing that you could measure would be the absolute levels of this neurotransmitter, dopamine, which, by the way, is originated from the amino acid tyrosine.
You could sort of spot some of the parts of that. That would be the carbon. Becomes a c-- there's a carboxylate and an amine. Oh, I've drawn the alcohol. This should be an NH2. Sorry, I just added this at last minute.
So dopamine actually though originates from tyrosine. So I just thought you would be interested because when I talk about the highlighting things that are in the news,
I mean things like this where we're all kind of interested. It's cool. It's relevant to what we do. And it really combines the efforts of scientists and engineers to make tools and methods and invent methods to make measurements that are quantitative enough to guide the analysis of a disorder.
So I just want to wrap up a little bit on the aspects. I was showing you the energy diagrams in the last class and telling you how enzymes affect the course of a reaction by lowering the energy of activation, by stabilizing a transition state or a high energy intermediate state. So the energy of activation becomes smaller in the catalyzed reaction, therefore faster than any uncatalyzed reaction.
And I did not give you this number last time. Enzymes catalyze reactions through about 10 to the 6, a million 10 to the 10-fold. So these are dramatic increases in rates that are really physio-- we depend on physiologically. They ensure specificity. And they're essential in all systems.
And the way we discuss energetic changes in reaction diagrams is by looking at what's known as the delta G. It's the change in free energy.
What I show you here is an exergonic reaction, where it is a negative delta G. I didn't reinforce that point enough last time. The energy of the products is lower than the energy of the substrates. So energy is given out at the end of the transformation.
That means the reaction is favorable with respect to an equilibrium, a thermodynamic parameter. And then it's the enzyme that takes care of the kinetic aspects of the reaction.
So just to sort of show you the correlate, an endergonic reaction would look like this, where the delta G is positive. The products are less stable than the starting materials, which would mean the reaction is not favorable. But it will still proceed in the presence of a catalyst.
And in this next small bit where we talk about pathways and different aspects of metabolism, I'm going to tell you how we get around the unfavorable equilibrium problem. Because that's obviously a big predicament in biochemistry. If reactions aren't favorable, why do they go far enough to be useful to us in metabolism?
So that reaction would have a positive delta G. And then I also mentioned these two terms. Anabolic refers to the endergonic reactions. I don't know why you're doing this to me-- the endergonic reactions. And catabolic refers to the exergonic reactions.
OK, then this last point-- I showed you this slide last time. But I think it was important to think about, why are enzymes so big? And I want to give one example of an additional genetic mutation that causes a human disorder, where I at least show you how the mutations are spread quite a distance from the reaction center to show you that all of that structure that you see in an enzyme as it interacts with a small substrate is critical for catalysis.
So we see small substrates in each case. But we have this very large enzyme, which is many, many times its size, all engaged in catalysis. But what's the proof of that?
So why are enzymes so big? So phenylketonuria is a human disorder. It's one of those disorders that neonates, brand newborns are tested for. They are checked for the genetic signal that shows that the protein will have mutations in its structure. And there are many mutations associated with a defect in this particular protein.
And the protein that I'm talking about is phenylalanine hydroxylase. So the disorder is related to defects in phenylalanine hydroxylase.
Now what does that enzyme do? It takes phenylalanine and installs a hydroxyl group opposite to where it's attached to the amino acid. So this is the hydrophobic amino acid phenylalanine.
And this is another one of the hydrophobic amino acids in a similar family that's tyrosine. And in fact, it's the precursor to dopamine physiologically.
Now it turns out there can always be too much of a good thing. So if you have too much phenylalanine, it has to be converted to a different amino acid. Because the build up of phenylalanine gets to a certain stage where it is converted itself to a toxic byproduct that actually causes severe mental disorders and seizures.
So the body needs to monitor the levels of phenylalanine. And at a certain stage, phenylalanine hydroxylase will convert it to tyrosine. So even though phenylalanine is essential, too much phenylalanine is a bad thing.
So that enzyme is the one that is associated with defects-- with mutations. The lower the activity of the phenylalanine hydroxylase and end you up accumulating phenylalanine too high. And so the PAH regulates the clearance from the body converting it to tyrosine.
So why-- you know, I told you I would give you some insight into how entire enzymes are in fact critical for cat lysis. So what I'm showing you on this a little movie is that the sites shown in magenta all around this protein, the active site would be where this big ball is. It's actually cofactor iron.
But the sites that are involved in the reduction of activity of phenylalanine hydroxylase are way out on the protein, out on its perimeters. So this protein is about 49 angstroms, 4.9 nanometers across.
But the sites that cause a reduction in activity are a long way away, 10 angstroms longer, 15 angstroms, 16.
So it turns out that enzymes as catalysts don't just use the local environment right near where chemistry happens. The entire protein collaborates to make the changes happen in catalysis.
So enzymes are big, because you're not just using this inner shell of functional groups where the substrate binds. You're actually using a lot of the dynamics of the enzyme to promote catalysis. And just this sort of visual shows you how far away things can be where they suppress the activity of a protein and make it a poorer catalyst. OK.
So as I said, this is one of the disorders, the genetic mutations that is tested for at birth. If you have one of these set of mutations, you immediately have to be put on a diet that's low in phenylalanine, so you don't bombard your body with too much phenylalanine, not allowing the phenylalanine to build up too much.
But I don't know if any of you have lately grabbed a can of Coca-Cola that's got aspartame as a sweetener. It turns out that sweetener, NutraSweet rather, is a dipeptide. See? But it contains phenylalanine.
So if you drink a ton of diet drinks that include NutraSweet, you are actually bombing yourself with high levels of phenylalanine that the body can't deal with. So people who have phenylketonuria, i.e. a defect in that enzyme, shouldn't be drinking or using NutraSweet type sweeteners. Because they actually give you too much phenylalanine at once, that you can mitigate the levels of.
So I want to just tell you about these genetic disorders. There's a fairly decent set that are tested for at birth. But the ones that are tested are for ones that you can make dietary modifications or lifestyle modifications and mitigate the symptoms. And so that's a very important thing to know.
You're not tested for things you don't know how to fix, because that wouldn't be appropriate. All right, any questions about that? Yeah.
STUDENT: [INAUDIBLE]
PROFESSOR: Yes.
STUDENT: [INAUDIBLE]
PROFESSOR: Oh, you know, that was me being clunky with-- of course you guys notice everything. This morning, I was making-- all right, let's go forward.
One, two, and that and that. I know exactly what you're talking about. This little curb down, yeah that's me shaking when I'm doing the ChemDraw drawing. It should be flat.
But thank you for noticing that. Just so that's not ambiguity, there should not be-- there could be, actually, a little dip when substrates bind to enzymes. But I didn't mean to imply it.
OK, anything else? OK, so what I want to talk about now is the equilibrium problem. And what is that?
So if we have reactions that are endergonic-- so we have one of these situations where we have a substrate going to a product that's higher energy. What that means is, at equilibrium between substrate and product, which is defined by the delta G, you have substrate going to product. But mostly, you've got a lot of substrate there, which is the amount of each of these is defined by this energy difference. OK.
So how do we survive with these kinds of transformations when we really want the flux for an enzyme to be in the forward direction? Because if we need the product, we need to move things forward.
So nature deals with this by coupling reactions to other reactions. So it finds ways around the equilibrium problem by, for example, putting a series of reactions.
For example, let's go from A to B to C. So these are three intermediates catalyzed by enzyme 1, enzyme 2. OK.
And let's just say this has an unfavorable delta G. So we don't make-- we have a lot of A. We have very little B.
And then what happens? How are we going to-- and then let's just say, for example, this reaction is favorable.
So let's see. The delta G here is positive. The delta G here is negative. So by plus VE, I mean positive and negative.
So this is a favorable reaction, whereas this is an unfavorable reaction. How does putting two reactions adjacent to each other happening on the same substrate and moving through help that situation?
Yeah.
STUDENT: [INAUDIBLE]
PROFESSOR: Perfect. So the answer here is that, as you take whatever B you have and turn it into C, A has to-- A1 has to make more B. So you solve the equilibrium problem in that way to a certain extent by just opening the tap at the other end of the series.
And nature organizes a ton of reactions in sequential pathways to get around these kinds of problems. So this is done by coupling reactions.
And so there are some reactions that are highly favorable, highly exothermic, where you can really sort of guarantee flux through that enzyme. A lot of these enzymes are ATP dependent. They use and burn ATP. So you get a lot of energy out.
And they drive the flux through enzyme one, by enzyme to being an exergonic reaction, while the enzyme one is and endergonic. So flux is guaranteed. And a lot of pathways sort of set up to be in this way with this coupling of the chemistries.
What else? So what we find is that enzymes, first of all, work in pathways where they are co-located in certain places. They may be, for example, co-localized to certain organelles in a human cell. They may be co-localized in the mitochondria or in some organelle. And we'll talk more about organelles and mitochondria later.
They could be co-localized at a membrane. And so you ensure that the enzymes are together by putting them in the same place. And then nature also evolves ways where the enzymes physically interact with each other, either covalently or non-covalently.
So they could be associated in the pathway by some kind of non-covalent interaction. Or you may actually link enzymes to make them single species.
So if this is enzyme one and this is enzyme two, here you have an enzyme one, enzyme two single long polypeptide chain that has two domains. They can't get away from each other, because they're joined covalently. And they catalyze sequential reactions.
This just doesn't happen with just three enzymes. It can happen with 10 enzymes or more. So there are very clever ways to ensure the flux occurs through pathways.
OK. But don't forget, all along, that each of these enzymes is responsible for a single transformation. That's important.
There's another phenomenon that having flux through pathways is very useful for, is to deal with toxic intermediates. Because that makes it very advantageous, again, to couple reactions.
So let's say product B is toxic. We don't want it hanging around much. We don't want it released from an enzyme to go do damage somewhere in the cell.
So having flux through a pathway basically ensures the B never gets out of the game. It just goes straight to enzyme two. So that's another physical advantage of those processes being linked together.
And finally, the process also ensures a very nice opportunity to do regulation. So here we on this slide, I show you this sort of mega mess of metabolic pathways in physiology. That there would be the Krebs cycle. But all the metabolic pathways that are all interlinked. And many of these pathways will be co-locali-- steps in these pathways will be co-localized as clusters.
And so that deal tells you how we can solve the equilibrium problem by linking enzymes-- the flux through pathways. And one of the best examples is in aerobic glycolysis, where in the early steps, they're not energetically favorable. You use ATP to start to break down glucose. Then you get to a certain intermediate where it's conversion to a smaller molecule generates ATP with very favorable reactions. So glycolysis is a really great example of this.
Now the other thing that I just want to describe to you is the issue of feedback. And I've described this here on this slide. And so we'll talk about it on this slide.
So if we are working with a pathway that goes through multiple steps to make an end product, five steps, three steps or whatever. And you've made too much of that. You've already made a lot of that product. And you don't need anymore.
Nature also has in place a regulatory mechanism to feedback and stop flux through the pathway. You don't just stop all the enzymes. You find a way to stop the first enzyme.
So this is a very important paradigm in biochemistry. And that's called negative feedback.
So I'm showing you it here just in a very simple cartoon form in the isoleucine biosynthesis pathway. That's one of the hydrophobic amino acids. This is an intermediate that is on its way from this amino acid that is polar but not charged, threonine.
So threonine gets converted to isoleucine. We need threonine to make isoleucine. But once we got a lot of isoleucine, it binds to the very first enzyme in the pathway and acts as an allosteric regulator that dampens activity.
So in this case, I just want to point out to you that I'm showing a very common way we notate things. When we are talking about inhibiting a reaction, we draw it like this.
We align and then align to the so the arrow, see which means you've stopped the activity. So you'll see this again and again. You're going to see it a lot in signaling pathways, because things often feedback.
So another thing that nature ensures, in addition to not building up toxic products and dealing with equilibria, is that you don't want a ton of enzymes working to make something you don't need anymore. So you might as well take the end product and use it to stop the first enzyme.
As that in product becomes scarce, it dissociates from the first enzyme. And it turns the pathway back on again. And I think that's a really neat way of making that happen.
And also, in these cases, the enzymes have to be clustered as a group. Because you wouldn't get those local concentrations to be so advantageous if the enzymes weren't co-localized. So does that make sense? If they weren't in a really near location, the enzyme that present produces isoleucine, that isoleucine couldn't bind back to the first enzyme in the pathway if they were in different compartments of the cell.
OK, everyone good with that? Good. All right. OK, so now we are moving on to carbohydrates.
It's a pretty strange word, carbohydrate. But actually, it relates to early findings that glucose is a carbohydrate. And its molecular formula is C6H12O6.
So they called it a hydrate of carbon before when they knew the elemental composition but they didn't know anything about the structure. There's a lot of carbohydrates that don't obey this rule. But that's where the name comes from, a hydrate of carbon.
All right now carbohydrates account for-- what did I have here? 25% of the mass of macromolecules, so a good amount. They are very, very important, carbohydrates in central metabolism. We use carbohydrates as a source of energy. But also, carbohydrates are a part of storage of energy in the form of polymeric structures, that I'll describe to you.
There are different ones in plants and in humans. One is glycogen. One is cellulose.
But carbohydrates have an increasingly important role in the extracellular matrix in these polymers that are wrapped around your cells and also as signaling entities both inside and outside the cell.
So we used to think of carbohydrates and straight away connect this with metabolism. But the story is far greater than that. And I'll try to explain to you why. And it's because of the richness of functional groups in a carbohydrate.
So the simplest carbohydrate, before I go up there, is a three carbon molecule. This would actually be called glyceraldehyde. But don't worry about that other name for it. It's a three carbon carbohydrate.
And this molecule would be called a triose. So we've got a couple of new-- a new suffix. Because anything that is a carbohydrate ends with the suffix "ose," not to be confused with the suffix "ase," which is an enzyme. So look carefully whether it's an "a" or "o," because it's the difference between a big protein that catalyzes a reaction and a carbohydrate.
So a triose would be a carbohydrate with three carbons. They have an aldehyde. Or let's just say they have a carbonyl functionality.
So remember, there would be lone pair electrons on those OHs, similarly on these. And remember, that each of these vertices corresponds to a carbon.
It's some-- the way you recognize carbohydrates is most commonly-- is that they are rich in carbon dash OH bonds, which are hydroxyls. Which makes them polar molecules, likely to be highly solvated in water and very different for from the compounds that are rich in just CH bonds that don't have such opportunities.
So if you see a molecule and it's got a bunch of CHs but not a bunch of OHs, it's probably a lipid. If you see a compound that's rich in OHs, it's likely to be a carbohydrate.
The story gets a little bit more interesting as we move up to some of the different carbohydrates, which I've shown you there on that screen. And I'm going to go forward to talk about those.
Because this triose is important in primary metabolism. We break down carbon carbohydrates that have six sugars to small carbohydrates that have three-- excuse me, carbohydrates that have six carbons to carbohydrates that have three carbons. And that's where these three carbon entities crop up.
But I want to focus you in on two sets of carbohydrates, the hexoses and the pentoses. So we immediately know they're carbohydrates, right? "Ose."
The hexoses obviously have six carbons. And the pentoses have five carbons.
And these are the most important of the carbohydrates. Yes, there are carbohydrates with four carbons. And then there were ones with seven, eight, nine carbons. But these are the ones will totally focus on in 7016, because these are very important in different biopolymers.
And the hexoses are important components, for example, of cellulose and glycogen. But where are the pentose carbons? And why are they so important?
Actually, I need to draw this is its straight chain version. Because I'll drive you crazy.
So this sugar here is a pentose, one, two, three, four, five carbons, a bunch of OHs, aldehyde at one end. Commonly, carbohydrates will fold up into a cyclic structure through an equilibrium. I won't worry you too much with the chemistry. But I'm just going to show you that structure.
It looks like this, a five-membered ring. The interconversion of these two is an equilibrium process. And those carbohydrates are incredibly important where?
Yeah, nucleic acids. So your phosphodiester backbone is attached to sugars that are attached a bit to purines and pyrimidines. And we'll see those structures later.
But an absolutely essential feature of that polymer is the five-membered ring carbohydrates that are known as ribose, which is what that guy is, and two deoxyribose, where one of the hydroxyls is actually a hydrogen. It's not a hydroxyl. And it's this one.
So instead of being an OH, it's an H. And we number carbohydrates. And I'll reinforce this much more when we talk about carbohydrate, about nucleic acids. There's a numbering system.
So two deoxyribose is in your DNA. And ribose itself is in your RNA.
OK, so obviously, we need to worry about carbohydrates and learn a little bit about them based on that key criterion. OK, let's move now to the hexoses.
I'm not going to make you keep drawing hexoses and things. I'm going to just tell you a little bit about them with respect to their structures.
So I've shown you on the board the cyclic form of a pentose. This is the linear form and the cyclic form. Let me write that down.
And by the way, in your DNA, it's always in the cyclic form. It's not in the linear form.
And the hexoses also have a linear and a cyclic form. And I show you that equilibrium for glucose. You see six carbons. I've got them numbered. And they form favorably into the six-membered ring.
This is the cyclic form of glucose. This is the linear form of glucose.
When glucose associates into polymers, it becomes these things like cellulose or glycogen. And it depends a lot on the linkages in those polymers to define which one it is.
Now, as I mentioned, carbohydrates aren't always just a series of OH groups. There's sometimes other functionality.
So I'm just going to quickly draw those. And if you've got them on your handouts, you can play along with me here.
So sometimes, there's an NH2 there. That's called glucose amine, very creatively. Sometimes that NH2 is converted to an amide, like that bond in a peptide. So it's glucose amide.
And sometimes-- so you can draw these on your handout, because the six-membered rings are there. This stays as an OH. But this OH here is at a different oxidation state. Don't worry about that terminology.
But what's important, it's glucuronic acid. So it can be negatively charged, positively charged, neutral. So there are variations on a lot of our hexoses, basically meaning this carbohydrate molecular formula doesn't work anymore. But the term has stuck.
So there's quite a variety of different carbohydrates with slight differences. And the intriguing thing is that the carbohydrates that you and I use in all of our physiologic processes are much simpler than the carbohydrates that bacteria use. There is an expansion of like 10 to the 2 or 10 to the 3 in the variety of sugars that bacteria use. And that's definitely a story for another day.
So let me now move on to thinking about carbohydrates not as the monomers that you metabolize, but rather as the polymers that are involved in many other different types of processes. And when we think about the polymers, we want to think about how their polymers differ from the polymers of nucleic acids or proteins. Because this will tell you why carbohydrates are so complicated.
When you take a bunch of amino acids and make a polymer, it's a linear polymer. Every unit is joined to the next by a name. There's no branching there. It's just a linear polymer.
So the diversity is not enormous. Or-- it's pretty enormous. But it's not as big as it could be if it were a branch polymer with different types of side chains.
We will see next week that the polymers of the nucleic acids-- here's the basic structure. There's the ribose, by the way. The R could be an OH or an H attached to a base of purine or pyrimidine-- you don't need to know that yet; you'll know it next week-- and then to a phosphate.
But those again, are linear polymers. You don't have branching. You just have a single continuous chain.
The crazy thing about sugars is they can branch from any of those OHs. So there's much more diversity of structure and function wrapped in the carbohydrates, which makes them real trouble to study.
OK, I want to now introduce you to another feature. The other thing is, when we join amino acids or we join nucleic acid building blocks, nucleosides, there's no difference in the shapes that we can form. We don't have variety there.
But in sugars, we can make different kinds of linkages depending on how two OH groups in a sugar are joined. So for example, if you join two glucoses, in this kind of linkage, that would give you maltose. But if you join two glucoses in that kind of linkage, it would give you lactose.
And those are different compounds. They serve different types of physiologic roles.
And there are enzymes that will make these bonds and then enzymes that will break these bonds. There is a common disorder that people have as they grow older. The enzyme that breaks the lactose bond the gets turned off. It doesn't work anymore. And that's the enzyme, lactose.
So when people are intolerant to sugars-- lactose is the sugar in milk. And they can't digest dairy products. Because lactase doesn't work anymore. Or you can take supplements. So that's how that relates to physiology.
The reaction between two glucoses to make the disaccharide is actually a condensation. Because when you join that bond, you kick out water as a side product. Or when you break that bond, you release water.
So this is another one of the condensation reactions. So underline that on the slide. Because condensation means a reaction that precedes and produces you a molecule of water.
And this just sums up the lactase problem, where you can digest lactose, the milk sugar. OK, so those are monomers.
Now let's think about polymers and complex structures of sugars. And I put these all on the slides, because it's just impossible for you to keep drawing them.
And I'm just going to give you sort of one of my pet peeves. I draw sugars like this. A lot of other people draw sugars like that.
And I don't like that. Because this is what they look like. So if you see this and you go, I haven't seen sugars looking like that before, it's because this is the way to draw them that actually represents their shape.
So if it's unusual format to you, I'm not going to ask you to construct these. I just want you to be familiar with looking at them like this rather than like this. Because, to me, that's the best way to render them.
OK, so polymers of sugar. I just mentioned to you, there are polymers of sugars that are important to storage. So when we have excess glucose, we store glucose as glycogen. It's often stored in the liver.
And later on in the semester, we'll see how a bolt of adrenaline sets all the processes in motion to chew up glycogen, to release more glucose so you can have a lot of energy quickly. So in that polymer, the sugars are linked in a different way.
The common polymer in plants and, in fact, accounts for a massive proportion of the biomass, is a different polymer of glucose where the linkages are beta-- we would call this a beta linkage. But that's the way it looks. And that would be cellulose. And it's a linear polymer.
Coming down here, glycogen is often a branched polymer with different kinds of linkages with different aspects or different shapes. So the glycogen that we store and can break down in order to produce glucose to make energy is glycogen.
We cannot break down cellulose. We don't digest plant cellulose, because we don't have those enzymes, which is why we don't get nutritional value out of the cellulose the same way we can put forces in action to break down glucose. So the way those bonds look is absolutely critical for how you can use the energy that's within them by using enzymes to break those bonds.
OK, so in general-- I've just said that. Glucose can be stored a cellulose or as glycogen. The process of photosynthesis converts energy and sunlight into glucose. And then you can make the polymers of glucose.
And what I'm showing you here in these polymers is a simplified view. I want to introduce to you one other term. And that term is glycan. And that basically refers to something that is more than one sugar, one carbohydrate.
I was looking at the videos of my lectures. I realized my handwriting is horrible. So I'm really trying very hard to make it a little bit neater today. So glycan is just the name for a polymer of sugars. But they can also be called polysaccharides. But glycan now is the commonly accepted term for a lot of sugars.
Now, I told you about energy storage. I've told you about simple disaccharides and monosaccharides and metabolism.
But what I want to do now is give you a little overview of all the different places where sugars feature in a cell. Because I think it's really important to realize that sugars form a great sort of set of molecules for communication.
So I'm going to go around this sort of funny looking square cell. And this would be a eukaryotic cell, because it's got a nucleus. And there are different compartments.
So within the cell, the cell sits in what's known as an extracellular matrix. It's a mesh work of sugar polymers that actually is important for the cells and important for trapping signals that come from cells and go to other cells. So those are all predominantly made up of carbohydrate.
The next thing to look at is that there are proteins within the cell. They can become modified with a sugar and go to the nucleus or leave the nucleus. So there is a type of signaling that is based on adding a sugar or taking it off a protein. We'll see later in the semester how also phosphorylation does a lot of functions that look like that.
There are sugars that are displayed on the cell surface polymers. And that's where signaling becomes important. Because sugars may be attached to lipids.
You remember, we've talked about phospholipids being part of the membrane. Sugars can be attached to lipids that sit in the membrane and face out. And it's how cell-cell communication occurs in some instances. Or they may be attached to proteins that are also displayed on the surface of the cell.
So this tells you a little bit about where we put these sugars is what's responsible for communication. Because they're on the surface of the cell with their sides out.
And then the final thing I want to talk about is the blood group system. So a lot of you may be aware of blood groups. There are four principal blood groups.
The differences between the blood in a A, B, AB and O blood groups are differences in just the sugars that are attached to the surface of the cell. So let me describe those. And then we'll talk about blood groups and things that are being done to enhance the supplies of red blood cells in cases of emergency. I have a nice little video I'm hoping to get to.
So on the surface of the cell, you might have different sugars. This is a trisaccharide.
All right, you can see one, two, three sugars. They have different linkages. But you can pick out the sugars. And they are joined by a bond called a glycoside bond and those join sugars.
So what do you think the enzyme that cleaves the glycoside is?
STUDENT: Glycosidase.
PROFESSOR: Glycosidase. You would say glycosidase. I would say glycosidase. But if they are the same thing. And that's going to be pertinent in a minute. So remember, if in doubt, guess and stick "ase" at the end if I ask you the name of an enzyme, because that works pretty well.
So if you have O blood group, you would have exclusively this trisaccharide on your red blood cells. If you have the A blood group, you have an extra sugar attached to that trisaccharide. And it's a glucosamide sugar. There's an extra carbonyl group on an amide.
And if you have B blood group, it's gluco-- it's a sugar. It's actually called galactose. That has all OH groups.
So the differences between if you're O group, if you're A group, or if you're B group, adjust those differences in sugars. And then people who have AB blood group have a composite. They have a mixture of the A and the B blood group.
So do you-- yeah, question.
STUDENT: [INAUDIBLE]
PROFESSOR: That's a second marker, which we won't talk about. So it's A plus another marker that's either one type or another type. But the AB and O are defined by the sugars. Yeah, good question.
OK, so do people know their blood group? It's good to know, frankly. Because someone-- you know, you may you cut yourself. And someone says, do you know your blood group? And you'll go, yes. And they'll give you an on-site transfusion.
I've been watching too much Jack Bauer. But, you know, that's a story for another day as well.
So it turns out that depending on blood groups, you can either be a universal donor or a universal acceptor. So the people with the O blood group can give anyone their blood. People with O, A, B, and AB. But unfortunately for the poor people with O blood group is that they cannot receive blood from blood group A, B, or AB.
And I wanted to show this. And I hope I can get it working. Because we have just the right amount of time, which makes me really happy. Because I don't really program things that well sometimes.
There was a pay-- there was-- the latest American chemist--
NARRATOR: --or other disaster. Those who are affected by crisis usually need four vital things, food, shelter, water and blood. In particular, O-type blood, because it can be safely given to any patient.
Now, scientists say they have identified enzymes from the human gut that can turn type A and B blood into type O up to 30 times more efficiently than previously studied enzymes.
Type A or B blood has specific sugars on the outside of its cells.
PROFESSOR: I just described--
NARRATOR: These sugars are recognized by the immune system. And if they don't match the type of blood that's already in an individual, those cells are destroyed. Because these sugars are recognized by the immune system, they're called antigens. Type AB blood has both antigens. And type O blood has none.
The researchers presented these findings at the recent American Chemical Society National Meeting in Boston. Stephen Withers from the University of British Columbia has been studying enzymes that remove A or B antigens from red blood cells. If those antigens can be removed, then type A or B can be converted to type O blood.
To find the enzymes more quickly, Withers and a colleague at his institution used a technique called metagenomics, which allows scientists to sample the genes of millions of microorganisms without the need for individual c--
PROFESSOR: So that tells you something about technology and engineering and understanding structures. So it would be really advantageous.
The stage at which you really need blood supplies is to take all the blood in the blood banks and turn everything into a universal donor. But the blood banks keep these differentiated supplies of blood.
But what if you need something that you can give everyone safely? And so there are enzymes in the gut. There are bacteria in the gut that actually live off of sugars that they chop off cells in the matrix in the GI system.
And so what the Withers group did was to screen a lot of different enzymes from bacteria in the gut and find ones that had a really good specificity for removing those sugars. Then they did protein engineering work to make them more efficient, thus creating something that was proposed a long time ago as could be useful, but now really making it useful.
Because those enzymes are much more efficient. They really catalyze reactions at great speed. So they're much more efficient engineering enzymes to treat your blood cells, to make sure you get rid of all the features that are characteristic of the A, the B, and the AB antigens.
Because if you don't do a good job of it, then the human immune system will recognize the little bits that sneak in and start triggering an immune response. So the efficiency of the enzymes that cleave those sugars off is absolutely paramount.
And that's what I have for you today. I will see you Monday, so don't forget. The Pset's due at 3:00. Monday, I would like for you to have a preview of the-- whoops, come on-- of the DNA reading material.
I don't know if any of you do this. But I think it's really handy. So there's just some very little sections that will give you a bit of exposure to nucleic acids. It'll just make the lecture a little bit more kind of familiar. But that's what we're up for Monday.