Flash and JavaScript are required for this feature.
Download the video from Internet Archive.
Description
Professor Martin begins his lecture on electrical signaling by talking about neurons, followed by action potentials, synapses, and optogenetics.
Instructor: Adam Martin
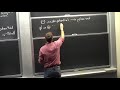
Lecture 22: Neurons, Action...
ADAM MARTIN: All right, let's get started. So I'm starting with this video here. What's happening here is there's this mouse, and you see there's like this fiber optic cable going into its brain. And the mouse is asleep right now. And now the researchers are shining light into its brain, a specific region of the brain, to activate specific neurons in order to test whether they function in arousal.
And here, you see the mouse is going to wake up. There it goes. It's awake now. So for today's lecture, we're going to work towards understanding how this experiment works. And we're going to talk about how neurons function and how researchers are able to control that function in order to modify behavior-- in this case, the arousal of this mouse.
OK, so this is going to involve a particular type of cell in our body, which is the neuron. And neurons are highly specialized cells that have a function to transmit information from one part of the body to another. And so neurons are highly polarized cells, which you can see here. On the left of this neuron, you see this arbor of protrusions, which are called dendrites. And then on this side of the cell body, you see a single extension, which is an axon, and then the terminus of the axon over here.
And this nerve cell transmits information in a single direction. It will transmit information from this side to this side. And these neurons are able to communicate with each other. And they communicate at the ends of the neuron, which are known as synapses, which I'll come back to and talk about later on in the lecture. So neurons could be making synapses on this side and also making synapses on this side with other neurons.
So to start to unpack the function of this neuron-- and I should highlight that this flow of information can occur over very long distances, right? Your sciatic nerve extends from the base of your spine all the way down into your foot, OK? So that axon is one meter in length. So that's an extremely long distance to transmit information along a single cell.
And so we're going to go from thinking about how signals are transmitted in single cells, and this will evolve electrical signaling. Then we'll talk about synapses and how synapses function to communicate between neurons. And this is going to involve also sort of understanding how certain antidepressants, like Prozac, work. And then we'll end by talking about how researchers did this experiment to wake up the mouse.
And it all starts with something that I told you about at the beginning of the semester, which is that the plasma membrane separates distinct compartments the outside of the cell from the cytoplasm. And there are distinct ion concentrations on either side of this boundary. So we're starting now talking about a single neuron cell. And we're going to talk about a type of signal known as an action potential. Oh, that's right.
So we're going to talk about an action potential. And what an action potential is, is it's an electrical signal that travels the length of the neuron. So this action potential, I'll abbreviate this AP. So when I refer to AP, I'm not referring to advanced placement, but action potential, OK? So this is an electrical signal that travels the length of the axon and the neuron.
And so in order to have an electrical signal propagate, we need some sort of electrical property that the cell has that enables this. And so I showed you or I told you earlier in the semester how sodium ions are concentrated on the outside of the cell and potassium ions are concentrated on the inside. You see here's the sodium gradient here, potassium gradient here. And now I'm going to tell you how it is that this happens, because this is thermodynamically not favored, right?
These ions would prefer, by diffusion, to be equal concentrations on both sides of this plasma membrane, which means that the cell to shift this from equilibrium has to expend energy to set up this situation. And so in the plasma membrane of the cell, there is a protein. It's an integral membrane protein and sits inside the plasma membrane. So this here is the plasma membrane.
And this integral membrane protein is called a sodium potassium ATPase. So it's going to have a subunit that hydrolyzes ATP to ADP. And the protein uses the energy of ATP hydrolysis to pump sodium ions up their concentration gradient. So the sodium ions are going out of the cell. And this is going against the flow that sodium would normally like to take, which would be going downstream.
And it pumps potassium ions into the cytoplasm such that there's a higher concentration of potassium ions in the cytoplasm, OK? So these neurons expend a huge-- a quarter of their ATP is used by pumping ions like this, such that there is gradients of ions across the plasma membrane.
Now, if one sodium ion was pumped out for every potassium ion pumped in, there'd be no charge difference between the exterior and the cytoplasm. But what happens in the plasma membrane is that in addition to the sodium potassium ATPase, there are other channels that are present. There are sodium channels. These are mostly closed, but there are some potassium channels that are leaky. And they're basically leaking potassium from the cytoplasm out into the exoplasm, OK?
And if you have positive charges going out the cell, then the inside of the membrane is going to have a net negative charge. And the outside of the membrane is going to have a net positive charge. And this charge across the membrane, where you have positive on the outside and minus on the inside-- I should label this exterior, and this is cytoplasm.
This voltage difference is known as a membrane potential. So this is a membrane potential. And it's an electrical potential across the membrane. If you're an electrical engineer, you can think of the plasma membrane as a capacitor, OK? So this plasma membrane is holding this charge difference across it. And so there's a voltage across the membrane. And in a resting state, the cell's resting potential is negative 70 millivolts.
So if the cell is not getting stimulated by something like a neurotransmitter, the resting potential is negative 70 millivolts, where the inside is negative and the outside is positive, OK? So now I just want to define some terms that are going to be useful for us when we talk about action potentials. So when there's this negative inside potential, a negative inside potential is referred to as polarized. So it's polarized because there's a polarity across this membrane, where one side is positive and the other side is negative, OK?
So polarized refers to if there's a negative inside potential. So the resting state of the side is there's a polarized-- it's polarized. However, the cell can lose this polarity and not have a charge differential, or it can flip and be positive on the inside. And when that happens, if there's either zero or positive inside potential, this is referred to as depolarized.
Anyone have an idea as to how the cell would flip the potential? What would have to happen in the plasma membrane to flip this potential and depolarize the cell? Yes, Stephen?
AUDIENCE: You could open the ion channels.
ADAM MARTIN: So Stephen suggested opening ion channels. Which ion channels would you open?
AUDIENCE: The sodium channels.
ADAM MARTIN: Yeah. So Stephen suggested if you open these, it's going to depolarize the cell. Because remember, sodium is high on the outside, out here. And so if you open these channels, positive ions are going to flow in. And that's going to make this less negative and this less positive, OK?
So this is the situation here, where these sodium channels open, and the sodium channels-- or the sodium ions rushing in is going to create a depolarization, where you now flip the potential. And there's a greater positive charge on the inside of the plasma membrane. Everyone see how? Because the sodium ions are going to just go downstream. They're higher concentration out here. So just by opening these channels, the cell doesn't have to do any work to do this. Sodium is just going to flow down its gradient into the cytoplasm.
So what an action potential is, is it's a transient depolarization of the nerve cell. So the Action Potential, or AP, is a transient depolarization of the neuron, which means it doesn't just depolarize and stay depolarized, but it depolarizes and then restores itself back to the resting polarity. And so what you see when you measure the voltage across the plasma membrane in a neuron, you see that it can spike and depolarize, but then it's rapidly restored to its resting state, OK? So it's a transient process.
When we think about the neuron at higher resolution, what you're going to see is not only is it transient, but it's also a traveling wave that propagates along the entire length of the cell. So this is also a traveling wave. And one thing that you can notice about these neurons, or the action potentials here, is that they all depolarize to the same extent. So they all depolarize to this positive 50 millivolts.
And so this illustrates a key property of neurons, in that the level of activity of a neuron is not determined by the size of this action potential. This action potential is an all-or-nothing event. It either happens or it doesn't. And when it happens, it depolarizes to the same level. So the action potential is all or nothing. You can think of it as a binary signal.
And therefore, the way that neurons encode sort of the magnitude of activation is not through the level of depolarization of a single action potential, but it is able to distinguish between different frequencies of action potentials that are propagating along the neuron. So signal strength, in this case, is related to the frequency of action potentials firing.
So now we're going to unpack how it is a nerve cell fires an action potential and how it propagates along the entire cell length, right? In the case of the sciatic nerve, this has to happen across an entire meter, OK? That's a very long distance to propagate this change in electrical signal, at least for a cell. And so we're going to talk about the mechanism. And I'm going to start at the beginning, when this action potential initiates.
So we'll start at the initiation of the action potential. So how is it that this nerve cell is told to start depolarizing at the dendrites? Because there's going to be another neuron here, which is going to communicate to this neuron over here to tell it to start depolarizing. It does this at the location known as the synapse, which is basically sort of the connection between the two neurons.
And the way this process is initiated is similar to the type of signaling that you saw in the past few lectures, where you have a ligand and a receptor, OK? In this case, the ligand is going to be what's known as a neurotransmitter, which is a small molecule. And I'll show you some later on. And the receptor is going to be a receptor that binds to this ligand.
But in this case, rather than being something like a G protein coupled receptor or a receptor tyrosine kinase, the receptor is going to be an ion channel, OK? So the receptor is going to be an ion channel. And so you see one example in the slide up here, where here's a receptor. And these receptors are what are known as ligand-gated ion channels. In this case, it's a sodium channel.
So it's going to be-- whether or not it's open depends on the presence of the ligand. So if we take a neurotransmitter like serotonin, if it's not bound to the receptor, the receptor is closed. But if serotonin binds to the receptor, it opens up the channel, which can selectively let in a type of ion-- in this case, sodium. In this case, this is an activating channel, because letting in sodium is going to depolarize the cell, OK?
So this ligand receptor binding uses a ligand-gated-- there's a ligand-gated sodium channel. And it's this ligand-gated sodium channel which starts the depolarization. So that's how you sort of knock over the first domino, right? But then there has to be some mechanism to propagate this along the length of a very long cell.
And so I'll tell you this involves a different type of sort of signaling mechanism from what you're used to thinking about, because this involves a different type of an ion channel. And it's called a voltage gated. And I'll abbreviate voltage gated just VG. And in this case, it will be a sodium channel.
So what's a voltage-gated sodium channel? This is a voltage-gated sodium channel here. And you can see, in the resting state of the cell, this channel is closed. And it's closed because of this red rod structure that's positively charged. That's a positively charged alpha helix that is a part of this protein and is embedded in the membrane. But this alpha helix is positioned down towards the cytoplasm, because it's positively charged. And the cytosolic face of the plasma membrane is negatively charged, OK?
And the confirmation of this protein then depends on the charge across this membrane. Because when there is depolarization, that shifts the position of this alpha helix, such that now it shifts up towards the exterior face of the plasma membrane. And that opens the channel, which lets sodium ions rush in, OK? Again, sodium ions here, they're always rushing downstream. They're concentration gradient.
So in this case, whether or not this channel is open or closed depends not on the presence of a ligand, but on the membrane potential across the plasma membrane. So these voltage-gated sodium channels, they're opened by depolarization.
And then the question becomes, if you open these channels at the very end of the neuron, how do you get it such that this electrical signal moves unidirectionally along the neuron? So what leads to unidirectionality? Who's been to a sporting event lately? OK, good. You guys know the wave?
So we're going to do the wave. Once you to stand up, you're going to be tired, and you're going to have to sit down for a while. I'm going to be a ligand-- I'm a ligand-gated sodium channel, so I'm going to start things off, OK? You ready? All right, here we go. OK, that's basically an action potential.
So the way that this was unidirectional is once you stood up and did the wave, you then sat down, and you stopped doing anything. And so these voltage-gated sodium channels have a similar property. If we look at the next step in this, the sodium channel is opened by depolarization. And you see there's this ball of chain segment of the protein. You see that yellow ball?
Once the sodium channel opens, after about a millisecond, that ball sticks in the channel pore and blocks it, OK? So these sodium channels open to let in sodium ions, but then they're immediately inactivated after about a millisecond, OK? And so that enables unidirectionality. So this is what I'll call voltage-gated sodium channel inactivation.
And how this promotes a traveling wave of depolarization is that if we consider an action potential moving along this axon from left to right and if the sodium channels in the green zone are currently open, it came from the left, which means that all the sodium channels to the left of this green zone are going to be inactivated. So because they're inactivated here, there won't be further depolarization going to the left, but depolarization will have to move to the right. And you basically get this traveling wave. And it goes one direction, because if it came from somewhere, which it always does, then where it just was coming from, all those sodium channels, the voltage-gated sodium channels are going to be closed.
So this allows it to move in a single direction along the neuron. Also, once the action potential gets to the end of the neuron, it doesn't reflect back the other way in the neuron. This can only go one direction. So this provides unidirectionality. So it's this inactive or refractory period of the voltage-gated sodium channel which prevents the action potential from moving backwards.
Now, if you look at these action potentials in the cell, you see that they happen, but you don't just depolarize and stay depolarized. The cell body depolarizes and then repolarizes very rapidly. So there's an oscillation. So there has to be some way to terminate the action potential. So there's a termination or repolarization of the cell.
So there has to be a way for this nerve cell to rapidly restore membrane potential. And I want you to think for just a couple of seconds about what type of channel might you open to re-establish this polarity. What ion do you need to flow from where to where in order to get a net negative charge on the inside? Udo?
AUDIENCE: You need to move the sodium ions from the inside to the outside.
ADAM MARTIN: OK, you could pump the sodium ions out, and that's totally accurate. So that's going to require moving sodium ions up a concentration gradient, which is going to take energy and is going to be slow. So is there another option we could take advantage of here to repolarize? Rachel?
AUDIENCE: Move the potassium ions.
ADAM MARTIN: So Rachel has suggested to moving the potassium ions to the outside, which is how this is done. So remember, potassium is high in the cytoplasmic, low on the exoplasm. And therefore, if you have a voltage-gated potassium channel, that's going to cause a rush of positive ions out of the cell. And that will be able to restore the net negative potential on the inside of the cell.
So this termination or repolarization is the result of the opening of voltage gated, in this case, not sodium channels, but potassium channels. When do you think these have to open relative to the sodium channel? Should they open right with the sodium channel? Carmen's shaking her head no. Do you want to explain your logic?
AUDIENCE: Well, I mean, they both carry the same charge, so they wind up getting out at the same time [INAUDIBLE].
ADAM MARTIN: Exactly. So what Carmen said is if they open simultaneously, you have sodium flowing in. You have potassium flowing out. And that's not going to necessarily change the charge. So when would these have to open relative to sodium channels? Yeah, Carmen?
AUDIENCE: When it reaches that potential [INAUDIBLE].
ADAM MARTIN: So after it's depolarized, yeah. So this has to be delayed relative to the sodium channels, OK? So this has to be delayed relative to the voltage-gated sodium channels. Because if you're thinking about this traveling wave of depolarization, the depolarization is going to be high where the sodium channels are only entering. And then following that, you would have potassium ions getting pumped out and basically repolarizing the cell.
Everyone see how you sort of get depolarization with sodium rushing in, and then after that, you repolarize with the potassium getting pumped out, right? So here, you have a spike, and you complete the cycle. It can even get hyperpolarized, where it gets even more negative than it normally does. And then it eventually gets back to this resting potential of around negative 60 or negative 70 millivolts.
OK, so this has to happen fast. And I want to tell you about one process or property of neurons and another helpful cell that enables this to go extremely fast. And that is that there are these glial cells in your body and your brain that wrap around the axons of the neurons and basically function like electrical tape for neurons, OK? So they are these-- there's electrical insulation around the axons of these neurons. And this is provided by another specialized cell type called a glial cell. So this is by a glial cell.
And here are two examples of glial cells. There are oligodendrocytes-- and you can see how the cell is extending processes that wrap around the axons of these two neurons. Here's a Schwann cell over here, which again, wraps around the axon. And these cells basically form what's called a myelin sheath. So they form a myelin sheath around the axons. And that insulates the plasma membrane of the axon such that-- so here is an axon.
You have glial cells that are wrapped around, and it sort of forms like beads on a string. And so there are these gaps between the myelin sheath that are known as the nodes of Ranvier. So there are these nodes of Ranvier, which are gaps in the myelin sheath. And these nodes perform an important function for the neuron, because where the axon is wrapped, the membrane is electrically insulated.
And so the sodium ions-- or the sodium channels and potassium channels, the voltage gated ones, localize to these nodes. And when the action potential is traveling along the axon, because these regions where the myelin sheath is are electrically insulated, the axon potential doesn't just move continuously, but jumps from node to node, such that you are just opening the sodium channels at these nodes. And that allows the action potential to travel about 100-fold faster along the axon. And that's what allows your neurons to transmit these electrical signals from the base of your spine to your foot so rapidly.
So you get an increase in speed because the action potential is jumping from node to node. And one important reason to bring this up is because there is an important human disease that affects the electrical insulation in the myelin sheath here, and that's multiple sclerosis.
So we're going to unpack multiple sclerosis in a couple lectures. This is an autoimmune disorder. And so we're going to talk about immunity later in the semester, and we'll talk about how that happens. But for now, I just want to point out that multiple sclerosis happens when the immune system attacks this myelin sheath.
So in multiple sclerosis, the myelin sheath is damaged. And if you damage this electrical insulation, you greatly slow down these action potentials, and that has a significant impact on nerve impulses in the brain and throughout the entire body. And that's why multiple sclerosis is such a devastating disease.
All right, I'm going to start moving now to consider more than one neuron. So until now, we've just talked about how an electrical signal is sent along the length of one cell. And now we're going to start thinking about multiple neurons and how they connect and how neurons integrate information from multiple other neurons to decide whether or not to send an action potential.
And so if we consider this connection right here, there's a synapse right here. Here's a cell that's sending information and a cell that is receiving that information. When we're considering a synapse-- so if we consider a synapse, there's a cell that is sending the signal, which is called the presynapse. This is the sender cell. And there's a postsynaptic cell.
But you can have more than one neuron sending a signal to a neuron at a given time, right? So here, you have one neuron that's sending a signal at this synapse, but you might have another neuron sending a signal to a synapse on this part of the cell. And you could have another signal coming in here. And so this neuron will then have to decide whether or not to fire an action potential down its axon.
And the way that the neuron decides this is to integrate the signals. So there's a signal integration process. And what's important for signal integration in a neuron is whether or not the cell body-- whether the voltage increases above a certain threshold potential. So if the cell body doesn't increase-- if the voltage doesn't increase above this potential, there will be no action potential fired. But if the voltage increases above the threshold potential, then it fires the action potential and signals to a downstream neuron or muscle or another cell.
So here, it is the threshold potential in the cell body that determines whether or not an action potential is sent down the axon. And there are different types of signals that nerve cells can send. So there are different types of signals. Signals can be excitatory, meaning it will tend to depolarize the neuron. So there are excitatory signals, which result in depolarization.
For example, with serotonin, that opens the sodium channel, and that results in depolarization, so that's an excitatory signal. But there are other types of signals that bind to different types of receptors that are inhibitory. What might be a type of receptor that would inhibit this process of sending an action potential? What might an inhibitory receptor be to lower the chance that this action potential will be fired?
What if I told you it's an ion channel? What ion would you expect it might pass? Udo?
AUDIENCE: Potassium.
ADAM MARTIN: Potassium. Udo is exactly right, right? If it passes potassium, then it's going to make the inside more negative. And that's what's known as hyperpolarization. So receptors that result in hyperpolarization would have an inhibitory effect on this process.
And remember, if you're hyperpolarizing, then you could cause this to actually go down and get even farther away from this threshold potential, right? And if you have an activating signal and an inhibitory signal, they might cancel out, because one will depolarize and the other will hyperpolarize. So it's in this way a neuron is able to integrate signals coming from different neurons. And that influences whether or not it will send the signal to a downstream cell.
OK, so now we're focusing on what is the communication between one neuron and another. And this revolves around this thing that's called the synapse, which is basically the gap between the axon terminal of one neuron and the dendrites of a postsynaptic neuron. And so the way that multiple neurons communicate with each other are through a type of signal known as a neurotransmitter. And this is what initiates the signal.
So there's a signal initiation process at the synapse. Initiation. And this involves the presynaptic neuron secreting a neurotransmitter. So the signal, in this case, signals between neurons are called neurotransmitters. And as you see on the slide, these are examples of neurotransmitters. They're often derived from amino acids, and so they're small molecules. They're not the proteins that you often see with receptor tyrosine kinase ligands. This is a different class of signal.
So one example is serotonin. And if you look up at those, we'll find serotonin here. There it is. Here, you can see it's a derivative of tryptophan. So it's a small molecule, and it's able to bind to a receptor on the postsynaptic cell and induce depolarization.
And so neurons are-- the way that they communicate is-- neurons are a case of where luck favors the prepared. Neurons are totally prepared to send signals to each other. They have everything ready to go when they get word from upstream, and they're ready to send signals to the next cell. And that's because if we look at the synapse prior to an action potential, everything is ready to go. The cell has neurotransmitter, and it's packaged in these vesicles, and it's tethered to the plasma membrane, ready to be released.
So prior to the action potential, there are vesicles filled with neurotransmitter that are docked at the plasma membrane. I abbreviate plasma membrane PM, just so I don't have to write it out, OK? So these contain neurotransmitter, right? But you see in this docked vesicle, the neurotransmitter is in red, and it can't get out if that vesicle does not fuse with the plasma membrane. So these contain neurotransmitter.
But at this point, the vesicles haven't fused. But the vesicle's not fused. When should they fuse? In this system of neuron signaling to each other, when should the vesicle fuse with the plasma membrane? What should trigger the fusion process? Yes, Miles?
AUDIENCE: So after [INAUDIBLE] axon when it's time for the [INAUDIBLE] that's when the vesicles fuse.
ADAM MARTIN: Yeah, so Miles is exactly right. If we consider my diagram here, there's an action potential traveling along this axon. When it gets to the axon terminus, that should be the signal for these vesicles to fuse to the plasma membrane and to release neurotransmitter. So it's the arrival of the action potential, right?
So remember, in this case, serotonin is going to be in blue. If serotonin is inside my vesicle here, it's going to need to exocytose. And now the serotonin is going to be outside the cell, ready to bind to the receptor.
All right, so as Miles pointed out, you have an action potential. The fusion should be triggered by the action potential. In order to fuse, there needs to be some signal inside the cytoplasm to tell the vesicles to fuse. That signal is increased calcium ion concentration. And then when calcium concentration increases in the cytoplasm, that triggers the fusion of these vesicles. And when you get fusion, that's exocytosis, and the serotonin is now on the outside of the cell, where it can travel across the synaptic cleft and bind to a receptor on the postsynaptic neuron.
So this fusion is when neurotransmitter is released. Neurotransmitter is released here. And the way that this increasing calcium has to happen, when the action potential arrives at the axon terminus. So when it arrives in the axon terminus, there's depolarization of that part of the cell. And so there's a special type of protein called a voltage-gated calcium channel.
All these channels are very selective for different ions. So a voltage-gated sodium channel isn't letting in all of the ions outside the cell. It's selective to sodium. And this case, this voltage-gated calcium channel is just going to let in calcium. And then there's a mechanism that links calcium entry to vesicle fusion. And that's going to be shown here.
What you see on this docked synaptic vesicle is this calcium-binding protein called synaptotagmin that's present on the vesicle. And so when calcium goes into the cytoplasm, that protein binds to calcium, and it activates the fusion machinery such that the plasma membrane of the vesicle fuses-- or the membrane of the vesicle fuses with the plasma membrane of the cell, thus releasing the neurotransmitter into the synaptic cleft.
So this is what starts the signal. Now, you probably know that these neurons are not active or on all the time. So something has to terminate the signal, usually quite rapidly. So now I want to talk about that. So like all signaling pathways, signaling is useless if you can just turn it on. You have to be able to toggle it on and off in order for biological systems to function properly, right? And that's the case with neurons.
If you just turn on a neuron and you don't have a way to turn it back off again, then that's pretty useless. And so we have to have a way to turn off the signal. And if we consider the synapse, this is the presynaptic neuron here. I'm going to draw a postsynaptic neuron here. And neurotransmitter is released by the presynaptic neuron to the postsynaptic neuron here. Neurotransmitter is released into the synaptic cleft.
So the sort of extracellular region between these two neurons is called the synaptic cleft. So now the cell just dumped a whole boatload of neurotransmitter into the synaptic cleft, right? How is it going to turn this off? What does it have to do? Yeah, Stephen?
AUDIENCE: It could absorb the-- take back in the [INAUDIBLE].
ADAM MARTIN: Stephen's exactly right. What Stephen suggested is, is there a way for the presynaptic neuron to reabsorb this neurotransmitter and, thus, recycle it? So it could either reabsorb it or degrade the neurotransmitter. Different process for different neurotransmitters. For serotonin, there are channels that are present in the plasma membrane, and these mediate reuptake of the serotonin.
So you have channels that are basically-- after the neurotransmitter is released, it sucks the neurotransmitter back into the presynaptic cell such that it can then reuse the neurotransmitter later on. And so this process of reuptake highlights a very important process that's been utilized by drug companies to create antidepressants. So antidepressants like Prozac and Zoloft affect this reuptake process. And what that does is it keeps the neurotransmitter in the synaptic cleft for longer, such that it enhances the signaling.
And so the idea behind these drugs is that if you are suffering depression from a lack of serotonin, then you can rescue that by preventing the rapid reuptake of the neurotransmitter into the cell after the synapse is stimulated and the neurotransmitter is released. And so Prozac, Zoloft, these are a class of drugs that are known as selective serotonin reuptake inhibitors.
It's kind of a mouthful. This is abbreviated SSRIs. But the way they function is to leave the neurotransmitter in the synaptic cleft for longer so that you enhance signaling, even if you have low levels of the neurotransmitter to begin with.
I also want to point out that if we look at this diagram here, the synaptic vesicle fuses, and then this releases the neurotransmitter. But all the machinery on this vesicle is recycled by endocytosis such that it can be reused again, OK? So cells are really good at recycling stuff. If this is sort of the membrane, you endocytose and then you can use it again later on, OK?
And so there's recycling not only of the neurotransmitter, but also all of the machinery on the synaptic vesicles that are responsible for the fusion event. All right, now I want to end by just telling you how this experiment works, where we're able to activate specific neurons in a brain and that leads to the animal sort of waking up. So in a normal neuron-- so this is the last part, optogenetics. And I'm going to go through this very fast.
But normally, you need a neurotransmitter to induce depolarization. But what optogenetics is, is an approach to control the activity of a cell with light, OK? So in this case, we're going to have light inducing depolarization. And the way this is done is there's a protein discovered from photosynthetic algae that's responsive to light, and it is a sodium channel. And this protein is called channelrhodopsin, specifically ChR2. And this is a light-sensitive protein where light induces sodium channel opening.
So that's going to depolarize the cell. And what you can do is if you have a gene that you know is specifically expressed in a certain type of neuron, you can take the promoter and enhancer region of that gene and hook it up to this single component, channelrhodopsin, that open reading frame, using recombinant DNA technology. And if that's expressed specifically in the neurons that you're trying to test, you can then shine a light into the brain of the organism and activate, specifically, this type of neuron. And that allows you to test the function of the neuron in the behavior of an organism.
So, in this case, this mouse, the light is shined into its brain, and they're testing a specific type of neuron that is involved in arousal of the mouse, and it wakes up. Oh, it's not playing. So here, this is the brain activity on the top, and the muscle activity on the bottom. So you're going to see light. There's the light. You see it? Light going into the brain.
They induce light at that frequency for a while. And then they're going to wait and see when the mouse wakes up. And it's going to wake up right now. There it goes. It woke up. You see now its muscle activity is going, OK?
So you can test the function of specific nerve cells using this approach, and it's because you have a light-sensitive sodium channel. So I'm done for today. Have a great weekend. I will see you on Monday.