Flash and JavaScript are required for this feature.
Download the video from Internet Archive.
Description
Professor Martin continues with genetics, discussing the laws of inheritance, and how they connect to the behavior of chromosomes undergoing meiosis.
Instructor: Adam Martin
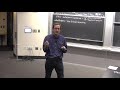
Lecture 13: Genetics 2—Ru...
ADAM MARTIN: So today, we're going to continue with genetics. And we're going to talk about the rules or laws of inheritance. I expect that many, if not all of you have been exposed to these rules before. And so what I really want to do today is make the connection between these laws and the behavior of chromosomes, such that when you're thinking about genetics and inheritance pattern you're thinking about chromosomes undergoing meiosis.
And I wanted to start just to make the point that there are a number of human traits and human diseases that show clear inheritance patterns. And what's shown up here is what's known as a pedigree. So this is a pedigree. Let's block this off. I'm showing you a pedigree, which shows relationships and a family tree.
And so what you can see-- what these symbols denote are, you have an open box. That is an unaffected male. So open box is an unaffected male. And what I mean by unaffected is usually it means they don't have the disease that we're talking about.
Circles represent females. And so an open circle would be an unaffected female. And finally, if you have one of these symbols, either male or female, and it's shaded in, that, by convention, represents an affected individual. So that's an individual that has the disease or trait. So this is an affected individual.
So this is an example of a disease that you've already heard about from Professor Imperiali. This is a family tree that's indicative of a type of inheritance that's seen in families with a disease called phenylketonuria. And you'll remember from Professor Imperiali's lecture, this is a disease where there's a mutation or allele in which there is a defective enzyme that can't process the amino acid phenylalanine. And so patients with this disorder have to be very careful about their diet such that they don't intake too much phenylalanine.
And what you see about this trait or disease is that it's skipping multiple generations. And then it manifests itself down here. I'm not getting any arrow. So I'm just going to use this pointer here. So here you see there are several individuals. There's a male and a female with the disease. And it results from a relationship between two first cousins. So only in this case does the disease sort of appear. And you can think of this as a recessive trait. In this case, it's autosomal recessive. So for PKU, this is exhibiting what's a type of inheritance known as autosomal recessive.
And the reason that it's recessive is because if an individual just has one copy of a functional enzyme, then they don't have the disease. So you can see that it's only individuals that have both defective versions, which are labeled lowercase a here, that exhibit the disease. So in the case of PKU, lowercase a represents the defective enzyme. And uppercase A denotes a functional enzyme.
Now, it's not shown here, but it's possible that an ancestor sort of above this parental generation also exhibited this disease, such that there'd be a sign that this is being inherited across generations. So that's not as obvious in this disorder, because it's a very rare genetic disorder. But more common disorders, such as color blindness, show a more clear inheritance from generation to generation.
So is anyone here colorblind? I ask just to know how to set up my slides as well. No one's colorblind. OK, that's good. Then you'll all see the difference between the image on the left and the image on the right. So if you have normal vision, that's what you see on the left from this fruit stand. But for those that are missing the red photopigment in your cone cells, you exhibit color blindness. And this fruit stand would look like the image on the right.
So this is a clear example of an inherited trait in humans. And this is an example of an inheritance pattern that would be similar to human colorblindness. And here you can see a clear example where you have an affected individual, an affected male here. That male has five daughters, none of which exhibit the phenotype or disease. But several of those daughters give rise to progeny, sons, that have the disease.
So in this case, you see this trait skips a generation. But essentially, the grandfather here has passed on the trait to his grandsons. And so colorblindness is a little bit different from PKU, not only in the fact that it's more frequent-- so it's about 10% of males exhibit colorblindness in the population. But also you see with PKU you had both a female and a male affected. And in this case, you're seeing a preponderance of affected males, which seems to be not random.
And so this colorblindness exhibits a different type of inheritance pattern, which is known as sex-linked recessive. And I'm going to come back to this inheritance pattern at the end of the lecture. Because it's actually this type of inheritance pattern which helped researchers about 100 years ago make the connection between the unit of heredity the gene and chromosomes. So we'll talk about another example of this type of inheritance pattern at the end.
So for today, what we're going to talk about is we're going to start with some of the basic laws of autosomal inheritance. And so we're going to talk about Gregor Mendel and his seminal studies in the pea plant. And then towards the end of the lecture, we're going to talk about sex linkage. And I'm going to tell you about work done in fruit flies, and specifically, their eye color trait, which led to the linkage between the behavior of genes and the behavior of chromosomes. So that's what we have in store for today.
So first, I'm going to tell you a little bit about what enabled Mendel's theory. I guess I could start over here. So what enabled Mendel's theory? And I presume that most of you have heard about Mendel before. So there might be a little bit of a reminder for you, but I also hope that we kind of make a very clear connection between Mendel's theory and the behavior of chromosomes.
So Mendel did his seminal studies using the pea plant. And one aspect of pea plant biology that was really essential for Mendel's theory is that you can both self-pollinate pea plants, meaning you can take the male gametes from a pea plant and mate it to the female gametes from the same pea plant. So it's entirely within the same plant.
So you can self-pollinate, meaning you do a cross-- you basically cross a plant to itself, which, obviously, we can't do with humans. You can't do with many organisms. Or you can cross-pollinate, meaning you take the male gamete from one plant, and you combine it with the female gamete from a different plant. And as we go through Mendel's experiments, you'll see how this was used to define the rules of inheritance.
Another property of the pea plants and something that Mendel took advantage of was he chose traits of pea plants that exhibited a very clear dominant or recessive phenotype. So he used visible traits, visible traits with a very clear dominance or recessiveness.
And if we go back to our example of PKU, you can see how this human disorder, the genes that determine this disorder-- the alleles have a very clear dominance or recessiveness. The dominant allele is often denoted with a capital letter. In this case for PKU, you we used capital letter A. Or in this case, the disease allele was lower case a. And that's recessive.
And if an allele has dominance, what that essentially means is that being homozygous for the dominant allele is equivalent to having just one copy of that allele. And so I think if you think of the PKU example, this is very clear. Because the disease phenotype results from a defective enzyme. So if you just have one copy of the enzyme that's functional, then the human can have wild type or normal function.
So in order to really lack function of this enzyme, you need to be homozygous for the non-functional allele. So you need to not have any normal copy of that enzyme. Because if you just have one copy of that enzyme, you're OK. Because you have an enzyme that's functional and will carry out that function in the cells of your body.
The last point I want to make is that Mendel did his experiments starting with what are known as pure breeding lines. So he started with pure breeding lines. And what I mean by pure breeding are these are lines that if you take these plants and just self-cross them over and over again, generation to generation, they will only give rise to plants with traits that reflect the parental generation. So there's basically no variation.
And you'll see in just a moment that another way to denote pure breeding means that for a certain trait you have an allele combination which is homozygous. So another way to think of pure breeding lines are these are plants that have a homozygous allele composition. Homozygous meaning that either they have two copies of one allele or two copies of the other allele.
I just want to make the point that Mendel had to overcome a number of hurdles in order to get these results. And Mendel is not exactly your sort of clear example of a success story. So Mendel applied to get a teaching certificate in university. And he failed out. And I'll quote one of his instructors who said, I quote, "He lacks insight and the requisite clarity of knowledge." So that guy feels stupid.
So next-- so Mendel did his experiments. And the significance of his work was never recognized throughout his lifetime. He did his experiments in the 1850s and '60s. He died in 1884. He died not knowing at all what the significance of his work was.
Because his work was then found again in the early 1900s by the likes of Thomas Hunt Morgan and others. And they made the connection between Mendel's laws of inheritance and chromosomes. And it was really then that Mendel became the father of genetics. Because then there was a physical model for how inheritance was working. But he died before that happened. So he didn't realize that he was the success that we now know him to be.
Another interesting story. He started his work with mice. He wanted to breed mice with different coat colors. But he got in trouble with his bishop, because his bishop didn't approve of him promoting sex among these mice. Luckily, his bishop didn't take a plant biology course. Because of course, plants also have sex.
But he had to under come a number of hurdles. But he took advantage of his garden. And I was talking to someone in my lab the other day. And she said, you know what's great about Mendel? He had a garden. And he just didn't put it on Instagram. So he used his garden to his advantage.
And so with his modest garden, he came up with what are now known as the rules of inheritance. And I'll start with Mendel's first law. So Mendel's first law is that every adult has a pair of genes for a given trait. And these are now what we refer to as alleles. And we now refer to them as genes as well. Mendel did not use the term gene. He just had these abstract sort of units of heredity.
So his first law states that, every adult has two sort of units of heredity that can be different. And that they split during the formation of the gametes. And the probability that a gamete will have a given allele or a given unit is equal probability. So what I hope you can see is that this law, which is stated up there, is a direct result of the segregation of homologous chromosomes during meiosis 1.
Mendel did not know that. But now looking back, we can see how this manifests itself. And so during meiosis 1, you recall that the homologous chromosomes here line up at the metaphase plate. And they line up opposite each other, such that some of the gametes will get the capital Y allele shown here. And the other half of the gametes will get this lower case y allele. So there's a 50% probability of a gamete either having one or the other.
And of course, in meiosis 1, this is referred to as a reduction or division. Because the homologues are split. And so the genetic content of the gametes are divided in half.
So Mendel's first law, the evidence for it were the results of what is known as a monohybrid cross. So Mendel did what is known as a monohybrid cross where he took pea plants that were pure breeding for two different traits. One was their pea color. So it's yellow peas versus green peas. And he made a hybrid where he takes a yellow plant that arises from yellow peas and crosses it to a plant from a green pea. And this is known as the parental generation.
And so the result of this cross is that all of the peas were yellow. So 100%. This cross results in 100% yellow peas. This is known as the first filial generation, or the F1. And that should indicate to you which of these traits is dominant. Because if you take yellow peas and you cross it to green peas, and you get yellow peas, that means that the trait for yellow peas is dominant. So for the rest of this, I will denote the gene allele that confers yellow peas as capital Y and the gene allele that encodes for green peas as lower case y.
And you see what I'm doing here is I'm putting the phenotype-- I'm describing the phenotype there, which is just what the trait is that manifests in the organism. But the genotype, which is the combination of alleles in the organism, I'm showing beneath the phenotype. And if these gene alleles are splitting during gamete formation and then recombining during the formation of a new plant, then, in this case, this hybrid plant is going to have one allele that's capital Y and one allele that's lowercase y. And because these two alleles are different, this situation is known as heterozygous. So this is a heterozygous plant.
You can think of pure breeding being analogous to homozygous. Because if you cross yellow pea plant to itself, you will only get back yellow peas. So it breeds true. You can think of a hybrid as being equivalent to heterozygous, because there are two gene alleles.
So then what Mendel did is he didn't stop there. He's self-crossed or self-pollinated these F1 plants and looked at the resulting seeds. And so in the F2 generation, what he found is that he got back both the parental phenotypes-- so 75% of the progeny were yellow, had yellow peas. And 25% had green peas. So there is this 3-to-1 ratio here.
Now, if we think about this in terms of Mendel's first law, where there's a segregation of these alleles during the formation of gametes, and there's an equal probability of having either one of these alleles-- if we think about this cross here, these plants, both the male and female side, are producing gametes that are either big Y or little y. So this would be the female here.
And so because they're separating, and there's a 1/2 probability of having either the capital Y or little y allele for the male-- and there's also a 1/2 probability of having either of these alleles for the female. So if you look at the possible combination of gametes that could give rise to the F2 generation, you have some that will be pure breeding yellow. And the probability here would be the joint probability of having this gamete and this gamete, which is one quarter.
You then have two classes here that have one copy of the dominant allele and one copy of the recessive allele. So these will have the same genotype. And these three will have the same phenotype. They'll all be yellow peas. And so if you add up the probabilities of all three of these, you can see that 3/4 will be yellow. So 3/4 of the progeny will have the yellow phenotype. And you can see that another quarter of the progeny have the chance of getting two copies of the recessive allele, and therefore will be green. So just by considering this as a probability problem, which is what Mendel did, you can explain the ratios of the progeny that Mendel observed in his crosses.
And I want to point out the parallels between this simple cross with peas and the inheritance pattern shown by PKU, or phenylketonuria. So notice here you have green peas in the parental generation. But green pea skips a generation and only appears again in a subsequent generation. So that's a lot like PKU, where you can see there's multiple generations that go by where the trait doesn't manifest itself. But then it pops up again in that later generation where you have inbreeding in this family. So there's a clear connection between the results that Mendel got and cases of human disease.
Now we're going to go on and talk about Mendel's second law. So Mendel's second law, which is often referred to as the law of independent assortment. And another fortuitous thing in thinking about Mendel's experimental design and setup which was fortuitous-- he didn't know it at the time-- he chose traits that actually were present on different chromosomes of the pea plant. So the traits didn't exhibit what is now known as linkage, where they're are physically connected on the chromosome. So this law of independent assortment can also be explained by thinking about how chromosomes behave during meiosis, where the alignment of homologous chromosomes at the metaphase plate of meiosis 1 is essentially random.
So if we take a look at this example here, you can see I've drawn one particular configuration for the chromosomes. And I'm using sort of one gene pair here and another allele pair here. And so if the chromosomes were aligned this way, then when they segregate during meiosis 1 you'd get two classes of gametes. Some that are capital Y, capital R. And another class that's lowercase y and r.
So that's one possibility. But what's equally probable during the alignment of chromosomes during meiosis 1 is that the chromosomes line up like this. So rather than having the dominant alleles all on one side of the metaphase plate, you have a dominant allele for one homologous pair on one side and the other dominant alleles on the other side.
So how they arrange, how these homologous chromosomes arrange during meiosis is totally random. And if they arrange like this, you'd get alternative types of gametes. You'd get gametes that are uppercase Y, lower case r, and lower case y, uppercase R. So this law of independent assortment can be completely explained by the behavior of the chromosomes during meiosis 1.
So now I'm going to take you through the experiment that illustrated this. And this type of experiment is what is known as a dihybrid cross. And so a dihybrid cross is now a cross where you're taking plants that differ in two traits rather than just one. So di stands for two. And in this case, we're going to consider both pea color. Again, the pea colors are yellow and green. But now we're also going to consider p shape. So you can have peas that are round and peas that are wrinkled.
All right, I'm going to make use of this board again. So let's consider the round wrinkled case. If you set up a cross between a plant that was from a round seed and a plant that was from a wrinkled seed-- and let's say the round allele is dominant, what would you expect to see in the F1?
So you have-- yes, Carlos.
AUDIENCE: All rounds.
ADAM MARTIN: You'd see all round, exactly right. So let's go through, now, this cross. So we're going to have a parental cross where Mendel took two pure breeding lines. One of them has yellow round peas. And he crossed the plant from a yellow round pea with a plant that was derived from a green wrinkled pea. And we already know yellow is dominant. And as Carlos just pointed out, if round is dominant, then you'd expect all of the peas to be round as well. So in the F1 generation, what Mendel found is you have 100% of the progeny that are yellow round peas.
And then similar to the monohybrid cross, Mendel self-crossed these F1 plants. And by self-crossing them, he observed a number of different classes of progeny. So he got back the parental types, yellow round. He also got back this other parental type, green wrinkled.
So these, because these were the same combination of traits that were present in at least one of the original parents, are known as perennials. They're parental. They had the same parental phenotype as one of the original parents. But what Mendel observed was two other classes of progeny which were different combinations of these traits that weren't present in the original parental generation. So those were yellow wrinkled peas and green round peas.
So you'll notice that this combination of traits, yellow and wrinkled, is not present in the parental generation. This is all F2. This is F2 continued. You also see green and round were not present in the parental generation. So these are referred to as being non-parental. So this non-parental class is a unique combination of traits that wasn't present in the original parents. And what Mendel noted was that in these dihybrid crosses he always got a stereotypic ratio of 9 to 3 to 3 to 1 for these different classes of combinations of traits.
Now we have to think about the probability. What leads to this characteristic ratio? And again, we can think about this just in terms of probabilities and these different gene pairs segregating independently of each other. So we already talked about for a monohybrid cross for pea color, 3/4 of the progeny is yellow. Because they at least have one dominant allele for color. And one quarter are green. So the probability of having this phenotype is 3/4. The probability of being green is one quarter.
And you can consider pea shape as just a separate monohybrid cross where the dominant phenotype is also going to be present at 3/4 probability. So 3/4 are going to be round. And one quarter is going to be wrinkled. So now if we just consider these different classes of progeny here, we can consider two monohybrid crosses. And what's the joint probability of being both yellow and round? So the joint probability of being yellow and round is 3/4 times 3/4. So if we have 3/4 times 3/4, that's going to equal 9/16.
Now, if we consider yellow and wrinkled, that's the joint probability of 3/4 and one quarter. So this probability is being 3/4 times one quarter, which is equal to 3/16. Green and round is similar. There's a quarter probability of being green and a 3/4 probability of being round. So again, you have one quarter times 3/4, which equals to 3/16.
And the least probable class is being homozygous recessive for both alleles. Because there's a one quarter probability of being recessive for each. So the joint probability of having all recessive alleles is one quarter times one quarter, or 1/16. So you could draw a massive Punnett square and also derive this. But really you can just consider it as two separate monohybrid crosses and then just calculate joint probabilities.
Any questions on Mendel before I move on? I'll just point out one thing about Mendel's second law. This is a rule that I'm going to break, now, in just a minute. And this law of independent assortment assumes that there is no linkage. In other words, seeing this type of inheritance pattern really depends on the two genes not being physically connected to each other on the chromosome.
Now we're going to talk about fruit flies. And specifically, we're going to talk about a certain trait in fruit flies, which is their eye color. I brought some pets to class today. And so we're going to talk about the white mutant phenotype, where the fruit flies have a white eye color. So I have three pairs of vials here. In one of them, there's the white mutant. And you're going to see it has white eyes. And then there's also a corresponding sort of normal red-eyed flies in the other vial.
So I'll just pass these around. Hopefully there's enough light that you can see the eye color. You're able to see the eye color, Jeremy? Yeah. You might have to come up to the board lights at the end of class if you want to see it really well.
So we're going to fast forward from Mendel now and talk about researchers who picked up on Mendel's work in the early 1900s. And specifically, I'm going to tell you about research done in the lab of Thomas Hunt Morgan, who had a fly lab at Columbia. So we're going to talk about Thomas Hunt Morgan. And actually, we're going to focus a lot on work done in Morgan's lab in the next couple of lectures. Because it turns out his lab also made the first genetic map. And we'll talk about that in Friday's lecture.
So the type of inheritance that Morgan defined is what is now known as sex-linked inheritance, where a given trait isn't a sorting independently of an organism's sex, but is somehow connected to it. And I want to sort of return your attention to this example of human colorblindness where there appears to be some sort of connection between the disease phenotype, in this case, colorblindness, and the gender of the individuals. So you see this disease is only affecting males.
And this type of inheritance pattern, while observed in humans, is really explained by work done in flies on this white mutant that you're carrying through the class. So sex-linked inheritance, the explanation for that is this is a trait that's carried by a special type of a chromosome known as a sex chromosome. So fortunately, for us, flies, like humans, have a similar set-- or male flies and humans have an X and a Y chromosome. So the inheritance kind of is similar between flies and humans when considering sex linkage. And females have two X's.
So the presence of these sex chromosomes was known in the fly. And it was known that if a fly had an X and a Y chromosome it would be a male fly. And if a fly had two X chromosomes it would be a female fly. And normally, normal flies have red eyes. But Morgan's lab was interested in variation in organisms. And they searched and searched for flies that had abnormal characteristics or traits.
And what they found in Morgan's lab was a mutant fly. It was a spontaneous mutant. But this fly had white eyes. And it was male. So they found a single white-eyed male, which they continued to study for some time. So they sort of defined some of the rules of its inheritance.
So what Morgan and his lab did was they set up a set of crosses that look a lot like Mendel's crosses. So you have a white-eyed male. They took a white-eyed male. And they crossed this white-eyed male to a red-eyed female. And if you cross a white-eyed male to a red-eyed female, the result was actually similar to what Mendel had predicted, which is that 100% of the flies had red eyes.
So that's similar to what you would expect from a monohybrid cross where red eyes is dominant. So I'm going to refer to the red eyed allele as an X with a capital R. And the white-eyed allele is an X with a lower case r. Because this gene is present on the X chromosome, but it's not present on the Y chromosome.
So the Y chromosome is really small. And so the X chromosome has-- all of its genes are basically present in one copy in the male. And that's going to manifest itself in that sex linkage.
So then they took this F1 generation where you have red-eyed males. And they crossed siblings. So they did a sibling cross. The one failing of flies as a genetic system is they can't self-cross. You have to have a male and a female. So they crossed two individuals in this F1 generation.
And what they found-- again, similar to what Mendel would predict-- is that 75% of the flies had red eyes. And 25% had white eyes. So this is behaving a lot like the yellow trait in peas. Except that all of the white-eyed flies in this F2 generation-- all of these white-eyed flies were male. So only the males were getting this trait of white eyes.
And you can see that that's very much reminiscent of colorblindness, where you have a grandfather that has white eyes. And the grandfather is essentially passing on this trait to his grandsons. So this pattern of inheritance that happens in the fly is very similar to that that happens in humans.
So now let's think about how this-- if we can explain this by thinking about chromosome segregating. So if we think about this F1 generation, we have red-eyed males-- or actually, let's do the parental cross first, where we have white-eyed males and we have red-eyed females. So all of the females are going to get their X from their dad. And they're going to get a wild type copy of this gene from their mom.
So all the females are heterozygous for the white gene. So I'm going to call it the white gene because that's what it's called. In flies, they name the genes based on the mutant phenotype. So if you mutate it, and it results in white-eyed flies, then they call it the white gene. All the males are going to have a functional copy of the white gene. And thus, all of these F1 flies are red-eyed.
So now these siblings are mated. All the females are heterozygous for this gene. The males all have a functional copy of the gene. So they're going to make gametes that are either an X that's functional for this eye color or the Y chromosome.
So now what you see is that all of the females are going to get this normal copy of the gene from dad, and thus, are going to have red eyes. Whereas half the males are going to get a functional copy from mom, and therefore, have red eyes. But the other half of the males are going to get this non-functional variant that can't produce red pigment, and therefore, are going to be white. So this here is your white class. And you can see because males only have one X chromosome, the only class of progeny that's going to be white-eyed here are those males that occur with a quarter frequency.
One thing I want you to think about over the next couple days-- and I'll sort of take you through it at the beginning of next lecture-- is what would happen if you set up a reciprocal cross here? What if you mated red-eyed males to white-eyed females? And I want you to tell me what you expect would result. So my question for next Friday is, what if you took red-eyed males and mated it to white-eyed females?
So I want you to think about this. And I want you to think how this is different from Mendel's experiment. What if you did a reciprocal cross for, let's say, the pea color? How would these two different crosses compare with each other? And we'll talk about that at the beginning of Friday's lecture. And we'll also talk about the first genetic map, which was actually created by an undergraduate. So stay tuned for that.
See you on Friday.