Flash and JavaScript are required for this feature.
Download the video from Internet Archive.
Description
Professor Martin introduces cell imaging techniques, which are tools that allow biologists to observe what's going on living cells. He goes over several types of microscopy and discusses how each deals with the challenges of resolution and contrast.
Instructor: Adam Martin
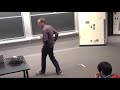
Lecture 29: Cell Imaging Te...
ADAM MARTIN: OK. So I'm going to start out today's lecture on the wrong foot by quoting somebody that you guys probably don't know and who was a New York Yankee. So Yogi Berra, the famous Yankee catcher once said, "You can observe a lot by watching," OK? And that is very appropriate for biology because a lot of things in biology have been discovered simply by watching for them in cells or watching for them to happen at the molecular level. And so our ability to visualize and see what's going on inside cells and at the molecular level is really critical for the process of biological discovery.
So today I'm going to tell you about tools, both sort of older tools but also kind of the cutting edge, for how biologists are really observing what's going on in living cells and in life in general.
OK. So let me start by just having you guys think a little bit. What do you require of me to see what I write on the board? Yeah, Rachel.
AUDIENCE: Light.
ADAM MARTIN: What's that?
AUDIENCE: Light.
ADAM MARTIN: You need light. And what does the light help you to do? What's that?
AUDIENCE: [INAUDIBLE]
ADAM MARTIN: You need it to see the board. And so let's say the light's on, OK? Is that, can you read this? No, what's the problem? What's that? Size, right? So Natalie suggested size.
Right, so one thing that you need is some amount of magnification, right? But let's take another-- let's say I do magnify this. What if I magnify it? And I'm going to start writing my notes on the board, right? How is this? Helpful?
Jeremy, what's wrong?
AUDIENCE: Differentiate [INAUDIBLE].
ADAM MARTIN: Yeah. You have to be able to distinguish different objects, in this case, these letters, right? So in addition to just magnifying it, you also need the structures to be far enough apart such that you can distinguish them. So you need what is known as resolution.
OK. This was resolution. But this is resolution where the letters are actually resolved, OK? So structures need to be far enough apart so that you can resolve them.
Now let's come back to Rachel's point, right? Why is it that we need light to see what's on the board? Right? What if I draw without pressing? Right? Is that-- yeah, Orey.
AUDIENCE: You need contrast.
ADAM MARTIN: You need contrast. Exactly, right? The light sort of gives you contrast between the chalk and the black part of the board. So you also need contrast. And contrast is the ability to-- the structures need to be differentiated from the background, OK? So structures need to be different from background.
What else do you need to read my writing? Right? What if I were just to-- everyone can read that? What's wrong? Carlos?
AUDIENCE: Needs to be clear and legible--
ADAM MARTIN: What's that? Yeah. I need to have, like, good handwriting, right? So I like to think of this as this is an aspect of sample preservation, OK? So there's a sample preservation issue. I can't butcher the letters and the words.
OK. So in the process of doing all these other things, right, magnifying your image, resolving things in your image, and generating contrast, you can't destroy your sample such that it's illegible, basically, OK? So in this case, structure must be preserved while doing one through three on this list.
OK. So I'm going to start with resolution. We'll talk about, what are the limits to resolving things in biological specimens. And in biology, the one instrument we use a lot is a microscope, OK? And a microscope is basically a collection of lenses that allow you to do many of the things I just drew on the board.
I'll point out a couple sort of broad sort of types of microscopy. So the human eye up here can resolve up to about 100 to 200 microns, if you're looking at something at reading distant distance, right? But cells are like way smaller than that, right? So we need some sort of instrument that allows us to see things that is lower than the resolution limit of a human eye. And so one way is to use a light microscope where you're using visible light to observe your sample. And many of the images that you're seeing that we're showing you are from visible light microscopes.
To see smaller things, type of microscope that's often used is an electron microscope. And electron microscopy allows us to observe structures all the way down to the sub nanometer level of resolution, OK? Now one limitation to the electron microscope is, you have to kill the sample, OK? So that can lead to artifacts and problems. And we'll discuss away at the end where light microscopy is being extended down to the limits that approximate that of an electron microscope.
OK. So what determines then, the resolution of a microscope? And so I'm going to sort of define a measurement of resolution which I'll call the d-min, or minimum distance. And this will be the minimum distance between two points that can be resolved.
OK. And what I showed you on that past slide is basically the limit on the right here is the d-min for these different types of microscopy techniques, OK? And what that means is, so the minimum distance would be, if your minimum distance is 200 nanometers, if two points are greater than 200 nanometers apart from each other, then you can distinguish them as two different objects.
However, if they are closer than 200 nanometers together, you wouldn't be able to see that these are two different objects. They would be overlapping each other, OK?
And typically, the d-min for a light microscope is around 200 nanometers, OK? And the d-min results, if we are to determine-- if I'm to tell you what determines this minimum distance, we have to think about a microscope. So here, I'm drawing a specimen here. I've just drawn my specimen. It's on a slide or a cover slip. Here's your specimen here.
And you might have a light source to generate the contrast. And then there'd be some sort of objective lens underneath the slide and the specimen. So this would be an objective lens. Sorry about my sample preservation here.
And so the light is going to be hitting the sample. And the objective lens will be collecting a cone of light that's going into the lens here, OK? And maybe I'll magnify this a bit so you can see it better. So I'm just going to magnify this region over here.
So if this is my specimen, I'm going to draw the objective a little farther away this time. This is the objective. And the objective is able to capture a range of different angles of light that come from the specimen, OK? So it's collecting angles. And I'll just define here an angle theta, which is like the 1/2 angle of this whole cone of light, OK?
So what determines the resolution limit in this type of system is first of all, the wavelength of the light that's used. So if you're using white light, that might be from 400 to 800. If you're exciting GFP, you're going to excite with a wavelength that's 488 nanometers. So it's usually around maybe between 450 and 550 nanometers for many different fluorescent proteins.
OK. So lambda here is the wavelength of the excitation of the light you're using. And this is all then divided by 2 times the NA, which is a property of the subjective. And what NA is, NA stands for numerical aperture. And what that is, is basically the range of angles that this objective can collect, OK?
So it's N sine theta, where theta is this angle here. So you get the best performance if the objective can collect all of the light that comes from this side of the sample. OK, and then N refers to the refractive index of the media that this light is going through.
OK. And so if you have an objective and you have your sample in here and there's a slide and a cover slip-- I'll extend this out-- you often have immersion oil. There'd be some sort of immersion media here.
And I don't know if you've ever used a microscope that's meant to be used with immersion media and you don't add that immersion media. But your image quality, if you don't add that media, is like really bad, right? And that's because you're affecting the numerical aperture of what this lens can collect. And therefore, you degrade your image quality, OK?
But basically, the more light, the more angles of light that you collect, the higher the numerical aperture. And therefore, the lower this d-min is going to be. And so the greater you'll be able to resolve objects that are near each other in space.
OK. So the take home message from all of this is that you notice that magnification is not a part of this. But the wavelength of the light is really critical, OK? So usually, this minimum distance ends up being the wavelength of light that you're using divided by 2. And this usually ends up being about 200 nanometers. So that's the diffraction limit of a light microscope, as you see up there.
And this resolution is basically limited by the diffraction or behavior of light, OK? So light microscopy is limited by the diffraction of light.
And it was thought for a long time that no matter what you do, you'd never be able to break this limit of about 200 nanometers. But at the end of the lecture, I'll tell you about some very smart people who figured out a way to actually break this limit. And we'll talk about how they were able to do that.
Now I want to talk about a few other limitations of microscopy. And I'm starting by showing you this electron micrograph of the endoplasmic reticulum.
And one important consideration you have to make is two dimensional versus three dimensional structure. So for electron microscopy, you basically cut the sample so you have a very thin slice of it. It's like slicing bread except these slices are on the order of 30 to 60 nanometers in height. And then you pass an electron beam through the sample after it's stained in order to visualize your specimen.
And one thing you have to keep in mind is that, you're looking at a slice through this. And it doesn't give you three dimensional information, OK? So if we were to think about the endoplasmic reticulum, you might have an endoplasmic reticulum. And if you take an optical slice through this, then you would see something like this where you see each of the stacks individually.
And so this might make you to conclude that the way that the endoplasmic reticulum is structured is it's kind of like a stack of pancakes, where each of these, you have a lipid bilayer surrounding a lumen of the ER. So right, the lumen would be inside like this for each of these. And they're just stacked on top of each other.
And this is the textbook model for endoplasmic reticulum structure. But it was actually, if you don't consider this in 3D, you might miss something. And what was missed was reported in 2013 in this paper, where rather than just taking a single slice, what they did is they made lots of slices. And they kept track of where they are. So they basically did a three dimensional reconstruction of the endoplasmic reticulum.
And by imaging this other dimension, they came to a very different conclusion about how the endoplasmic reticulum is organized. And instead of being stacks of membranes on top of each other like pancakes, instead, it's a helicoid. So this is an ER from a professional secretory cell, like a salivary gland cell. And you can see in 3D, you get a very different picture of the organization of this organelle. It's actually wrapped around and spiraling membrane stacks, OK?
So their model is that, basically the endoplasmic reticulum in some cell types basically has a parking garage like structure, OK? So in this case, in these cells, it seems like the ER Is basically a parking garage for ribosomes. OK. And you don't get that information unless you consider the three dimensional structure of the thing that you're looking at.
So in addition to electron microscopy, there are techniques that involve light microscopy that involve optical sectioning. And so normally, if you're looking at fluorescence, if you're doing fluorescence microscopy, you'd be exciting the whole volume of your sample and exciting all of the fluorophores such that fluorescence from out of the focal plane would be getting into your image. And that would give you a much more hazy, unclear image.
But there are techniques such as confocal fluorescence microscopy that allow you to exclude the out of focus light such that you're basically getting an optical section through your sample. And that can give you a much cleaner and better resolved image, OK?
Now I want to talk a little bit about another dimension, which is time. And again, you're seeing images in textbooks. And usually, you're just seeing a single image. And whenever you see a single image, you have to think about how things might be changing in time in order to understand the system.
So one example that I like here is shown here, where these are different proteins that are labeled in a yeast cell. And you see that these proteins form patches at the edge of the yeast cell. And some of these patches just contain the green protein, which is SLA1. And other patches contain just the red protein which is ABP1. And there's another class of patch which contains both, OK?
So how might you interpret this fixed image over here? What might be one model you would conclude? Well, what was initially concluded from this type of experiment is you have three different types of patches that are distinct from each other in the cell because they have different molecular compositions, OK?
And that was what was initially thought. But it was wrong because researchers had to really consider the aspect of time in this problem. And I'm going to show you a movie now over here where you're going to see this yeast cell. And now you have these different proteins tagged with different fluorescent proteins. And we can watch them in time as they progress through a stereotypic cycle.
So what you're going to notice in this movie is that you see these green patches appear. And every single green patch at some point is joined by red. And then the green disappears and the red stays around. And then the thing disassembles, OK?
So what was initially thought to be three different structures in the cell, eventually, it was found out that there was a dynamic process where this patch sort of matured over time and eventually disappeared into the cell. And what this process is, is actually endocytosis in yeast. And you're seeing different proteins getting recruited to endocytic vesicles as they bud from the plasma membrane of this yeast. OK. So that's just my caution in interpreting fixed images, because you have to think about how they might be changing in time.
All right. So now we have to consider contrast. And in bright field microscopy, and bright field microscopy basically involves white light as your light source. And so you'd have a microscope that has a white light source. You might have your specimen here. Here's your specimen.
Then you'd have some sort of detector at the end of your system. And there would be some objective lens in between, which I'm going to ignore for now. And so, for bright field microscopy, you're taking a sample and shining it with light. The light that doesn't go through your sample will go right through to the detector. And that's your background.
But some of this light, the light that's going and hitting your sample, could be absorbed or it could be refracted. And it's the refraction or absorption of this light which generates the contrast for bright field microscopy. So in bright field, native structures in the cell absorb or refract light. And this is what generates the contrast.
OK. So the images shown up here are bright field images of cells. And in each of these cases, there's no dye. There's no fluorescent protein. But you're able to see the outline of the cell. And you're able to see even individual organelles or structures within the cell that are interacting with the white light and generating contrast, OK?
So that's one way to generate contrast is just hope that whatever is native in your cell generates the contrast. But there are also-- you can increase contrast in specimens by adding dyes. And if these dyes bind to specific structures like a membrane, then that will increase your contrast.
So the electron microscopy images that I showed you-- so for electron microscopy, you generate contrast by adding a dye that is an electron-dense dye, which will bend the electron beam. And that's what allows you to get an image from an EM. So an EM contrast is from an electron-dense dye such as uranyl acetate or some other type of dye.
Now, fluorescence microscopy, as Professor Imperiali showed you, involves taking a fluorescent molecule and attaching it to your protein of interest. So you're actually getting protein-specific contrast, which is very useful.
OK? And the way a fluorescence microscope works is just shown up here where you might have a light source that has a range of wavelengths. And you can use a filter to select one. In the case of GFP, it would be blue light or 488 nanometer light. And that would then be shined onto your specimen. And then the light is absorbed by fluorophores in your specimen. Some energy is lost, such that the light that's emitted from GFP is a longer wavelength, in this case, green.
And then you can filter again to make sure only the green light is what goes to the detector. So this is a very efficient way of generating contrast because you can use filters to select only the wavelength of light that is emitted from your fluorescent molecule. OK. Any questions about that and about my very short version of how fluorescence microscopy works? Yeah, Rachel?
AUDIENCE: [INAUDIBLE] dichroic mirror?
ADAM MARTIN: The dichroic mirror reflects certain wavelengths that are below a certain wavelength. And will pass wavelengths that are above a wavelength. So it will basically reflect the excitation light. But it will pass the emitted light, OK? And so, there are tons of these mirrors. Some are not dichroic, but they can reflect four different wavelengths and pass all other wavelengths. And so, this allows you to image multiple fluorophores at the same time, OK? The specifics aren't as important as the general concept of how this works.
OK. Now I want to come back to the resolution limit. And I want to tell you about how we can beat it. So, beating. We all like winning. So beating the diffraction limit. And this is going to involve a type of microscopy that's really sort of been developed in the past decade, which is known as super resolution microscopy. So super resolution microscopy.
And remember, I mentioned for you before that yes, electron microscopy can get you nanometer resolution. But you have to kill the cell. And also, it's hard to get protein specific contrast, right? So that kind of sucks because as biologists, usually we're interested in how things are functioning to stay, to live. So wouldn't it be great if we could somehow use light microscopy to get down into this nanometer range so that we can see how individual proteins are interacting with each other and organized at the nanometer level, OK?
And so in the past decade, there's really been a revolution that's enabled us to do light microscopy with a resolution that gets down to the 10 or even single nanometer resolution.
And there's a number of different super resolution techniques. I'm going to talk about just one of them. But both these techniques basically use the same concept, which is that they enable whoever's doing it to identify single molecules and define where those molecules are very precisely. And to turn fluorescent molecules on and off so that you can select individual molecules such that you can see them.
So these are two different techniques. They're conceptually very similar. I'm going to focus on this one here. But it's pretty much similar to this one up here.
And I just want to point out that one of our colleagues here at MIT, Ibrahim Cisse who's in the physics department, his lab builds these super resolution microscopes. And they're using super resolution microscopy to study the collective behaviors of proteins, in his case, during the function of gene expression. OK, so this is research that's actively being pursued at MIT.
So let's just do a thought experiment again. OK. I'm drawing a single molecule or what you would see in an image if you were looking at a single molecule GFP. Great. Where is GFP here? Carmen?
AUDIENCE: It's right there on the board.
ADAM MARTIN: It's right there on the board, right? Is it here? What's that?
AUDIENCE: I don't know.
ADAM MARTIN: Who thinks GFP is right here? Who does not think GFP is right there? You have to be thinking one or the other. Yeah, Rachel.
AUDIENCE: [INAUDIBLE]
ADAM MARTIN: OK. So what Rachel says is that it's probably not right here. It's probably in the middle of this thing, right? And so if you're seeing a diffraction limited spot, you're going to get some sort of Gaussian of intensity, which I didn't draw well here. But it might be a little bit brighter in the center and drop off as you go towards the edge, right?
So if I were to take a image intensity profile along the line here, you'd see something that kind of looked like a Gaussian, OK? And GFP, if there's a single molecule that you're imaging, should be right in the center of this Gaussian, OK?
And so even though we're not seeing a spot, we're seeing a spot that its width here is diffraction limited. So this width is 200 nanometers. But if we can estimate where the molecule is in this region with nanometer precision, we could get a very accurate view of where this fluorescent molecule is, OK?
So it relies on certain assumptions. The first assumption is that you're assuming we can see single fluorescent molecules. So that we visualize single fluorescent molecules. And that we can then estimate with some amount of precision the location of the molecule based on this diffraction limited sort of image that we get. So then we have to estimate the location based on the image.
OK. And then our resolution is basically the error in fitting this curve, OK? So the error in the fitted position is equal to the standard deviation of this Gaussian. The standard deviation divided by the square root of the number of photons that you collected to get that image. So the square root of the number of photons.
OK. And I just told you in the beginning of the lecture that this standard deviation is limited by the diffraction of light. So the standard deviation is going to be around 200 nanometers, right? But if you collect a lot of photons, you can accurately figure out where the fluorescent molecule is here if you know that it's a single molecule. OK?
So the number of photons in a typical experiment is going to be around 10 to the fourth, OK? And so if 200 nanometers by 10 to the fourth, you're going to have sub nanometer resolution if you do the experiment right. OK?
So you really need to see fluorescent molecules, however, in order to do this, OK? And the real breakthrough came with the realization that you could combine this type of fitting to estimate the position of single molecules with a certain type of fluorescent protein where you can turn the protein on and off stochastically, OK?
OK. So we need one more component which is a photo-activatable fluorescent protein, in this case, the first one was photo-activatable GFP PA-GFP.
And PA-GFP is a fluorescent protein like GPF. It's genetically encoded. But when it matures, it's not fluorescent. It's in a dark state, OK?
So when it matures, it's dark. It has a dark state. And it starts out in this dark state. But you can turn it on. And you can turn it on with light. So that's where the photo activation is, because you're able to photo activate this fluorescent molecule. And you can photo activate with sort of UV light or 405 nanometer light.
And so that's not normally the excitation wavelength. But if you shine your sample with 405 nanometer light, it will convert some set of your molecules into the now fluorescent state, OK? So this then causes it to be fluorescent. And now it's going to be lighting up.
OK. And I want to thank Professor Cisse because he gave me the next slide which I think nicely shows how this technique works. So the way you can get super resolution is you can't be looking at all your fluorophores at once because they're not far enough apart and they'll all bleed together so that you get a bad image, right? So this would be your conventional diffraction limited image where all the fluorophores-- there's about 20 fluorophores here. And you can see, you can't see individual fluorophores and you can't see what that says, OK?
But if you take a divide and conquer approach with this, if you have a photo-activatable GFP, you don't need to look at them all at once. You can just look at three to start, OK? So now if you only activate a small subset and you ensure that you're activating it at a frequency such that they're well resolved from each other, then you can distinguish that there are three single molecules here. You can fit where they are. And now you know where they are with nanometer precision. So you know where those are.
And then you want to look at other molecules. And so you have to get rid of these. And so what you would do is to bleach them. And bleaching is to use light to basically damage the fluorophores and get it to no longer fluoresce, OK? So this process is going to involve an iterative photo activation followed by measuring and fitting the image so that you can basically determine where each single molecule is in your image.
And then ending with bleaching to get rid of the fluorophores you just turned on so that everything is now dark again. And then you repeat this process iteratively to collect all of the single molecules that you can, OK?
So in this case, we just got these three molecules. We would then want to bleach them so that we're now going to look at different fluorescent molecules. And we'll turn on a certain number of other fluorescent molecules. Here you see four. Here are two. They're a little close together, but you can see that there are two. Here are another two. They're close together, but you see two clear intensity peaks.
And so you can fit those four. Now you have four more molecules to make up your image. You bleach them, excite or activate five more. Here are five fluorescent molecules. You can fit those. Determine their positions. And you just do this iteratively over and over again till you get as many molecules as you can.
And at the end, you basically add all these images together to get the final super resolution image, OK? So this is an iterative process where the photo activation allows you to image single molecules such that you can see where they are with nanometer precision. And then you add them all together to get a super resolution image.
Here is an example of this in practice. And this is the storm technique which doesn't involve a photo activatable fluorescent protein, but involves organic dyes blinking. And the concept is basically the same. And here you see a conventional image of an axon. And it's labeled with this beta-II spectrin. And you see beta-II spectrin is continuous. And it's staining in this axon.
But if you look at the super resolution image, what you see is the beta spectrin actually has this repeated periodic pattern along the axon. And this is a cytoskeletal element that's basically present in rings up and down the axons of neurons.
And you can kind of think of this as like the axon is a vacuum hose, where you have these rigid sort of rings that are aligned all along the axon. But because it's repeated and you have intervening areas without much cytoskeleton, you can kind of think of it as a way for the axon to be both rigid but also flexible and maneuverable.
I just wanted to point out that several super resolution techniques were recognized in the 2014 Nobel Prize in chemistry. Eric Betzig on the left here developed the approach using the photo activatable GFP that I described to you.
And two others, Stefan Hell and W.E. Moerner were awarded it for other types of super resolution technique. If you get a chance, you should go to the Nobel Prize website and listen to Eric Betzig's Nobel lecture. He has a very interesting story. And part of it involved how he managed to develop this technique. And he actually developed it in the living room of his friend.
So this is actually one of the first super resolution microscopes. Here's the microscope and here you see-- I love this chair. But you see, you basically have this microscope in this guy's living room. So if you want to hear more about this story, listen to his Nobel lecture. He's a really funny guy and you get a sense of how science really works where you get this unemployed guy like building a microscope in his friend's living room and then wins a Nobel Prize.
And just one reminder to end today, remember your news brief is due this Friday, November 30th. If you need help on selecting a topic, please see a member of our staff, including Professor Imperiali or myself. And so, good luck with that. Thank you. I'm all set.