Flash and JavaScript are required for this feature.
Download the video from Internet Archive.
Description
In his third lecture on genetics, Professor Martin picks up from the last lecture on eye color in fruit flies, and then continues with Mendelian inheritance. He then talks about linkage, crossing over, and gene mapping.
Instructor: Adam Martin
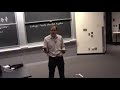
Lecture 14: Genetics 3—Li...
ADAM MARTIN: And so I wanted to start today's lecture by continuing what we were talking about in the last lecture. So I'm just going to hide this real quick. And so we're talking about the fruit fly and the white gene and the white mutant, which results in white-eyed flies. And we talked about how if you take females that have red eyes and cross them to males, the white-eyed male, then 100% of the progeny has red eyes in the F1 generation.
And so I asked you guys, would you get the same results if you did the reciprocal cross? So what if we took white-eyed females and mated them to red-eyed males? So what about this? Actually, I'm going to move this over to over here so that maybe it's more visible. So what if we have white-eyed females and crossed this to red-eyed males?
So let's unpack this sort of a little bit at a time. So what's the genotype of these white-eyed females here? Miles?
AUDIENCE: So if you designate the eye gene as the letter A, a female would be X lowercase a, X lowercase a.
ADAM MARTIN: Yes. So Miles is exactly right. So the dominant phenotype is red eyes, because the gene encodes for an enzyme that's important for the production of the red pigment. And so X lowercase a here would be a recessive mutant that lacks the pigment.
And because it's a recessive allele-- because you need only one copy of this gene to produce the pigment. So the recessive allele results in the white phenotype. Therefore, this has to be homozygous recessive. How about this red-eyed male? Yeah, Ory?
AUDIENCE: Wouldn't you have a Y and then an X capital A?
ADAM MARTIN: Yes. So this would be this phenotype, right, where capital A is the gene that produces-- is a normal functioning gene that produces the pigment. So then in your F1 here, are you going to see something similar to this or something different?
AUDIENCE: Something different.
ADAM MARTIN: Different, great. Who said different? Javier? Do you want to propose what you might see?
AUDIENCE: Yeah. For the males, they're going to inherit the Y gene from the father and the [INAUDIBLE].
ADAM MARTIN: Exactly. So the males are going to get the Y from the father, and they're going to get one X from their mother. So all the males are going to be of this genotype here, which means they're going to have what color eye? Javier is exactly right. That means they're going to have white eyes. So all the males will have white eyes. And what about the females?
AUDIENCE: [INAUDIBLE]
ADAM MARTIN: What's that?
AUDIENCE: Red eyes.
ADAM MARTIN: Yeah. So Ory is saying the males are going to get red eyes, right, because they're-- or the females are going to have red eyes, because they're going to get the X chromosome from their father, which has the dominant gene that produces the red pigment. So all the females are going to be heterozygous, but have a functional copy of this gene. So all of the females will have red eyes.
OK, does everyone see how-- now, how would this compare with Mendel's crosses and pea color? Would there be a difference in Mendel's crosses if you switch the male versus the female if these were autosomal traits? Ory is shaking his head no, and he's right, right? In that case, it doesn't matter. You can do the reciprocal crosses, you get the same result. But because this is sex linked, which one is the male and which is the female is relevant.
And this actually relates to something that we just saw on the MIT news. I just got this email this morning, but it came out I think yesterday, which is that biology-related research in the mechanical engineering department-- specifically, the CAM lab-- they've been able to design a 3D sort of model for ALS disease, which is also known as Lou Gehrig's disease. And so what they've done in the CAM lab is to take cells from either patients that have ALS or from normal individuals, and they coax these cells to become neurons.
Here, you're seeing a neuron in blue and green here. And you see the neurites extend from this neuron. And they have a model where this neuron can then synapse with a muscle. And so they're using this 3D sort of tissue model to model ALS and to look for drugs that might affect ALS, potentially curing ALS.
And so last night, I started reading about ALS and was pleased to find that there's actually a very rare X-linked, dominant form of the disease that can be passed on from generation to generation. And the inheritance pattern of this X-linked, dominant version of ALS would have an inheritance pattern that's similar to what we observe for the white mutant in the fruit fly, right?
Whereas, if you have an affected father, and this is a dominant mutant on the X chromosome, then all of his daughters will get that X chromosome and be affected, whereas the sons will all be unaffected. However, if you have the reciprocal situation, where you have an affected mother and an unaffected father, then the sons and daughters get the disease randomly. So this is a sort of form of inheritance, which is relevant if you're considering human disease and some forms of it.
Most versions of ALS, well, are sporadic, but inherited forms are usually autosomal dominant. So this is a rare case here. But I thought it was interesting in that it's relevant to what we've been talking about.
So now, just to recap-- here, I'll throw this up so everything's up. So in the last lecture, we talked about Mendelian inheritance. And we talked about when you take two parents that differ in two traits and you perform a cross, you get a hybrid individual that is heterozygous for both genes. And now this is the F1 individual.
Let's say we want to know what types of gametes this F1 individual produces. We can perform a type of a cross known as a test cross, where we cross this individual to another individual that is homozygous recessive for both these genes, which means that you know exactly which alleles are coming from this parent. And they're both recessive, so you can see whether or not the gamete produced by this individual has either the dominant or the recessive allele.
Let me see. I'll boost this up. So now we can consider the different types of progeny that result from this test cross. And some will have the two dominant alleles from this parent and will, therefore, be heterozygous for the A and B gene. And it would exhibit the dominant A and B phenotype. I think that is what I'm showing here.
So if the chromosomes, during meiosis I, align like this, then the two dominant alleles segregate together, and you get AB gametes. And you also, reciprocally, get these lowercase a and b gametes, as well. So that's the other class here. So you can get these two classes of progeny.
And the phenotypes of these two classes will resemble the parents, right? So these are known as parental gametes. So these are the parentals. But you know because Mendel showed that if you have genes and their alleles on separate chromosomes, they can assert independently of each other. So an alternatively likely scenario is that the chromosomes align like this, where now the dominant allele of B is on the other side of the spindle. And therefore, these chromosomes are going to segregate like this during the first meiotic division.
And that gives rise to gametes that have a different combination of alleles than the parents. So you have some that look like this. So each of these would be different classes of progeny. And you have one last class that would look like this. And so neither of these look like the original parents, and so they're known as non-parental.
And so if these two genes are behaving according to Mendel's second law, where there's independent assortment-- if you have independent assortment, what's going to be the ratio of parental to non-parental? Rachel?
AUDIENCE: One to one.
ADAM MARTIN: Yeah, Rachel says one to one, and I think a number of others also said one to one. So you have 50% parental, 50% non-parental, right? Because it's equally likely to get either of those alignments of the homologous chromosomes during meiosis I.
So now I'm going to basically break the rules I just explained to you in the last lecture and tell you about an exception, which is known as linkage. Gesundheit. And in the abstract sense, linkage is simply when you have two traits that tend to be inherited together. So just considering probability. So you have traits inherited together. They're exhibiting what is known as linkage.
But that's an abstract way to think about it. It's just based on probability, right? So a physical model for what linkage is, is that you have chromosomes. The genes are on the chromosomes. And for two genes to be linked, those genes are physically near each other on the chromosome. So the physical model is that two genes are near each other on the chromosome.
OK, so let's consider again these generic genes, A and B. If A and B resulting from this cross-- if these two happen to be on the same chromosome, now they're physically coupled to each other. Then they're going to tend to be inherited together. No matter how these align, they're always going to go together during the first meiotic division. And that's just going to only give you the parental gametes.
So if there's linkage, you're going to have-- let's consider the case where you have complete linkage. If you have complete linkage, 100% of the gametes are going to be parentals, and you're going to have 0% non-parental. That's if the genes are really, really, really close to each other, and maybe you don't count so many progeny. You won't see any mixing between the two.
But there is a phenomenon that can separate these genes, and it's known as crossing over. And another term to describe it is recombination. So the alleles are getting recombined between the chromosomes. Recombination. And what crossing over or recombination is, is it's a mixing of the chromosomes, if you will. Or it's an exchange of DNA.
So there's a physical exchange of DNA from one of the homologous chromosomes to the other, OK? So you can think of this as an exchange of DNA between the homologous chromosomes, OK? And that's important. It's not an exchange between the sister chromatids, but between the homologous chromosomes that have the different alleles.
And what's shown here is a micrograph showing you a picture of the process of crossing over. You can see the centromeres are the dark structures there. And you can see how the homologous chromosomes intertwine. And there are regions where it looks like there's a cross. Those are the homologous chromosomes crossing over and exchanging DNA such that one part of that chromosome gets attached to the other centromere.
So I'll just show you in sort of my silly cartoon form how this is, just to make it clear. So let's say, again, you have these A and B genes, and they're physically linked on the chromosome. During crossing over, you can get an exchange of these alleles, such as a bit of one chromosome goes to the other homologous chromosome and vice versa, OK? So now you have the dominant A allele with the recessive b allele and vice versa.
So now during meiosis I-- after meiosis II, this will give rise to two types of gametes, one of which is non-parental. And the same for the one down here. You get two types of gametes. One is non-parental-- the lowercase a, uppercase B gamete.
So this happens if there's incomplete linkage. That means there can be a recombination event that separates the two genes. And I'm going to give you an example of a case where data was collected with what fraction of each class there is. So now we're considering an example where you have a linkage. So A and B are on the same chromosome. And so we'll consider a case where, in this class, there are 165 members. For this one, there is 191.
So I'm kind of-- line down like this. And then for the first recombinant class, 23 individuals. And for the last, there are 21 individuals. So you can see there are many more of the parental class than the recombinant class, but we can calculate a frequency, or recombination frequency, between these two genes. And in this case, the recombination frequency is 44/400, which is equal to 11%, OK?
So 11% of the progeny from this cross had some sort of crossing over between the A and B alleles. It would've been up here. Now, this frequency is interesting, because it is proportional to the distance that separates these two genes. So this recombination frequency is proportional to the linear distance along the chromosome between the genes.
Now, it also depends on the recombination frequency in a given organism or in a given part of the chromosome. So when you're comparing recombination frequencies between different organisms, there's actually differences in the real different-- they're not equivalent. You can't compare them, basically. And also, there are regions of the chromosome where recombination happens less frequently than others. And so, again, you can't compare distances along those.
But overall, you can use this as a distance in order to map genes along the linear axis of a chromosome. And maps are useful, because you can see where stuff is, right? So in this example here, I'll highlight a couple of places. Here's Rivendell. Here's Lonely Mountain. Here's Beorn's house.
So let's say we are able to determine the distance between Rivendell and Lonely Mountain, and the distance between Lonely Mountain and Beorn's house, and the distance between Rivendell and Beorn's house. You'd be able to get a relative picture of where all of these places are in relation to each other. So this is a two-dimensional map I'm showing here. It's not one dimensional, but chromosomes are one dimensional, so it's a bit more accurate, OK?
So this idea that recombination frequency can be used to measure distances between genes and that this could be used to generate a map is an idea that an undergraduate had while working in Thomas Hunt Morgan's lab back in 1911. And what I find fascinating about the story is this guy basically blew off his homework to produce the first genetic map in any organism. So the person who did it was Alfred Sturtevant, and he was an undergraduate at Columbia working for Thomas Hunt Morgan.
And I'll just paraphrase this quote here. In 1911, he was talking with his advisor, Morgan, and he realized that the variations in the strength of linkage attributed by Morgan to differences in the separation of genes-- so Morgan had already made this connection, that the recombination frequency reflects the distance between the genes. But then Sturtevant realized that this offered the possibility of determining sequences in the linear dimension of the chromosome between the genes, OK?
So then-- this is my favorite part-- "I went home and spent most of the night, to the neglect of my undergraduate homework, in producing the first chromosome map." And this is it. So the first chromosome map was of the Drosophila X chromosome, which we've been talking about. There's the white gene, which we've been talking about in the context of eye color. There's a yellow body gene here. There's vermilion, miniature, rudimentary, right?
These are all visible phenotypes that you can see in the fly. And you can measure recombination between various alleles of these different genes.
All right, so now I want to go through with you an example of how you can make one of these genetic maps. And it's essentially the same conceptually as to what Sturtevant did. And it involves what is known as a three-point cross. So a three-point cross. So there are going to be three genes, all of which are going to be hybrid, and I'll start with the parental generation that is little a, capital B, capital D. And we'll cross this fly or organism to an organism that is capital A, lower case b, lowercase d. Yes, Carmen?
AUDIENCE: So when you write the gametes up there, does that imply that they were analogous parents?
ADAM MARTIN: So what I'm writing here is the phenotype, basically. And so these are homozygous for each of these, yes. I could also write this as-- but I'm not going to draw the chromosomes, because it kind of gets more confusing. I'll draw the chromosomes here in F1, because we have now, basically, a tri-hybrid with one chromosome that looks like this, right? They got that chromosome from this individual here. And another chromosome will look like this.
See? So this F1 fly is heterozygous for these three genes, and it has these two parental chromosomes. So now we can look at the gametes that result from this fly by doing a test cross, just like we did before. And so we want to cross this to a fly that's homozygous recessive for each of these genes. And now we can look at the progeny.
And just by looking at the phenotype, we're going to know the genotype, because we know all of the flies from this cross have a chromosome from this individual that has recessive alleles for each gene. So we can consider now this first one here. That's one potential class of progeny. Another class would be this one. And these two, you can see, resemble the parents, right? So these are the parental classes of the progeny. So this is parental.
All right, now you can consider all other combinations of alleles. And so I'll quickly write them down. You could have something-- progeny that look like this and this. These are just kind of reciprocal from each other. You could have progeny that look like this and this. And the last class would be this and this.
So all of these progeny that I drew down here are recombinant, because they don't resemble the parents, right? Because there are three genes, now there's many more ways to get recombinant progeny, as opposed to having just two genes, right? So you can have many different combinations of these different alleles. And so now I'm going to give you data from a cross with three such genes.
So you might get 580 individuals that look like this, 592 like this, 45 and 40, 89, 94, 3, and 5. So this is data that is, I believe, from fly genes. I've just ignored the fly nomenclature, because it's confusing, and just given them lettered names, OK? But this reflects data from some cross somewhere.
So now we want to know-- let's go back to our map. We want to make a map, OK? And so to make our map, we're going to want to consider all pairwise distances between different genes. So we'll start with the A and B gene. I'll write over here. So let's consider the A/B distance. And remember, to get a distance, we're looking at the number of the frequency which there is recombination between these two genes, OK?
So we now have to look through all of these recombinant class of progeny and figure out the ones that have had a recombination between A and B, right? So on the parent chromosome, you see little lowercase a started out with capital B and vice versa. So any case where we don't have lowercase a paired with capital B, there's been some type of exchange.
So here, lowercase a's with lowercase b. So that's a recombinant. Here, uppercase A's with uppercase B. That's a recombinant, too. So we have to add all these up. So 45 plus 40. How about here? Recombination here or no? Yes. I'm hearing yes. That's correct. Here, recombination, yes or no? Carmen's shaking her head no. She's exactly right.
So we just have to-- these are all the recombinants between A and B. So it's 45 plus 40 plus 89 plus 94, which equals 268 over a total progeny of 1,448. And that gives you a map distance of 18.5%. Because this method was developed in Morgan's lab, this measurement is also known as a centimorgan. It was named in honor of Morgan. So that's what I refer to when I have lowercase c capital M. That's a centimorgan. So you can also use centimorgan here.
All right, so that's A and B, but now we have to consider other distances. So how about the A/D distance? And again, we have to go through and figure out where the alleles for A and D have been recombined, OK? So little a is with capital D, and upper case A is with lowercase d. So we have to find all the cases where that's not the case.
Here, this is lowercase a with capital D and capital A with lowercase d. This is parental from the respect of just the A and D genes, but all the rest of these guys are recombinants, OK? So this is 89 plus 94 plus 3 plus 5, which comes out to be 191 over 1,448. And this is 13.2 centimorgans. So that's the distance between A and D. Distance between A-B, distance between A-D.
So the last combination, then, is just B and D. So if we consider the B/D distance, again, we have to look for all cases in which lowercase b and d become separated and uppercase B and D become separated. Here, they're separated. Here, they're separated. Wait, no, not here, sorry. Here, they're not separated. Here, they are separated.
Everyone see how I'm doing this? Are there any questions about it? You can just shout it out if you have a question? So this distance is 6.4 centimorgans. Everyone see how I'm considering every pairwise combination of genes and then just ignoring the other one and looking for where there's been a recombination in the progeny?
So now that we have our distances, we can make our map, right? So the two genes that are farthest apart are A and B, OK? So that's kind of like here, Rivendell and Lonely Mountain. Those are the two genes that are at the extremities. So I'll draw this out. It doesn't matter which way you put it. We're just mapping these genes relative to each other. But B and A are the farthest apart from each other.
Now if we consider the distance between B and D, that's 6.4 centimorgans. So it appears that D is closer to B than it is to A, because it's 13 centimorgans away from A, OK? So D is kind of like Beorn's house here. It's closer to Rivendell than it is from Lonely Mountain. So we'll put that in there. This distance is 6.4 centimorgans. And then the distance here is 13.2 centimorgans.
As far as I know, no one of the field of genetic mapping has been assaulted by large spiders, but the field is still young. So one thing that should be maybe bothering you right now is if you add up the distance between B and D and D and A, you don't, in fact, get 18.4 centimorgans. Instead, you get 19.6 centimorgans. This is 19.6 centimorgans. So it seems like, somehow, we are underestimating this distance here, OK? So we seem to be underestimating this.
So why is it that we are underestimating this distance? Well, to consider that, you have to sort of look at how all of these classes were generated. So now I'm going to go through each class, and we'll look at how it was generated. I'm also going to-- well, I'll just draw new chromosomes. So we have to draw this order now.
We have B, D, a. So the first chromosome is B, D, a. That's right, B, D, a. The other chromosome is b, d, A. So now we look and see how this recombinant class was generated. So this is lowercase a. We'll start with b-- lowercase b, lowercase a, but capital D. So it's capital D, lowercase a. So that recombinant results from this chromosome here, where there's crossing over, and little b gets hooked up to big D and little a. See how I did that?
And then if we consider this class, this is uppercase B, lowercase d, capital A. So these two classes of progeny result from a single crossover between B and D, OK? So this is a single crossover between B and D genes. And now we can go through and look at how this is generated.
So to get all recessive alleles on the same chromosome, there would be a crossover here. And so this is a single crossover between D and A. So a single crossover between D and A. Now, these last couple classes of progeny are interesting in that they're the least frequent class. And so when we consider how they're generated, we'll start with uppercase B.
Let's see if I can get rid of this. So uppercase B, lowercase d, lowercase a. And so what this last class is, is actually a double crossover. So this is a double crossover. And it's least frequent because there's a lower probability of getting two crossovers in this region. But now you see that even though it doesn't look like there was recombination between A and D, in fact, there was. There were two crossovers, and it just looks like there was no recombination, if you didn't see the behavior of gene D.
So if we take into account that there are actually double crossovers between B and A, then if we add that into our calculation here, where you add in 3 plus 5 multiplied by 2 because these are both double crossover events, then you get the 19.6 centimorgans that you would expect by adding up the other recombinations, OK?
How is that? Is that clear to everybody? You're going to have to make maps like this on the problem set and possibly the test. So make sure you can given-- yeah, Ory?
AUDIENCE: I realized that you immediately [INAUDIBLE] overestimated the difference between A and B and not overestimated B to D or D to A?
ADAM MARTIN: It's because when you have two genes that are very far apart, you can have multiple crossovers. And when you have sort of crossovers that are in pairs of two, then it's going to go from one strand back to the other, and so you're not going to see a recombination between the two alleles. So it's an underestimate, because if you have multiples of two in terms of crossovers, you're going to miss the recombination events. You see what I mean? You understand that you can miss the double crossover events?
AUDIENCE: Yeah, I get that.
ADAM MARTIN: Yeah, right? So then you're going to underestimate the number of crossovers that actually happened in that genetic region. All right, now I want to end with an experiment that, again, makes the point that genes are these entities that are on chromosomes. So just like you can have linkage between two genes on the same chromosome, you can also have linkage between genes and physical structures on chromosomes, like the centromere.
So you could have genes like A and B here that are present on the chromosomes and present very near the centromere of those chromosomes, OK? So they could be right on top of the centromere, OK? And to show you how this manifests itself, I have to tell you about another organism, which is a unicellular organism called yeast.
And yeast is special and that it can exist in both a haploid and a diploid form. So it has a lifecycle that involves it going both as a haploid and as a diploid. And so you can take yeast-- and we'll take two haploid yeast cells. And much like gametes, these can fuse to form a zygote. So, in this case, I'm taking-- again, we'll consider two generic genes, A and B. And we'll make a diploid yeast cell that is heterozygous, or hybrid, for A and B.
And what's great and special about yeast and why I'm telling you about this is because as opposed to flies and us and other organisms, the product of a single meiosis is packaged in this single package, if you will. So the yeast can undergo meiosis, and the product of a single meiosis is present in this case, where each of these would represent a haploid cell that then can divide and make many cells.
But this is the product of a single meiosis in a package, OK? So you can actually see the direct result of a meiotic division, a single meiotic division. So this is the product of a single meiotic division. And that's special because when we make gametes, we have individual cells. All the products of meiosis are split up, and then just one randomly finds an egg and fertilizes it. So you don't know which of the gametes are from the product of a single meiosis.
And so being able to see the product of a single meiosis allows us to see things like genes being linked to physical structures on the chromosome like the centromere. So if we consider this case, these two genes are both linked to the centromere. And during metaphor phase of meiosis I, they could align like this, in which case you would get spores that are parental for both dominant alleles or parental for both recessive alleles.
So each of these cells is known as a spore. So I'll label spore numbers here. So this is spore number. And in this case, you get two spores that are dominant for both alleles and two spores that are recessive for both alleles. Because there are two types, it's known as a ditype. And this is a parental ditype, because you have two types of spores . And they are both parental
An alternative scenario is that these chromosomes would align differently, right? So you get parental spores there. However, alternatively, you could have this configuration, where this is now flipped. And during meiosis I here, these chromosomes move together. And they, again, produce two types of spores, so it's a ditype. But in this case, all the spores are non-parental. So another scenario is you get this.
And because there are two types and they're non-parental, this is known as non-parental ditype. That's a non-parental ditype. And if these genes are linked to the centromere completely, then you can only get these two classes of packages, OK? So if these genes are unlinked-- so the two genes are unlinked, but both linked to the centromere, then you get parental ditype-- 50% parental ditype, type 50% non-parental ditype. So I'm abbreviating parental ditype PD and non-parental ditype NPD.
So what has to happen to get another type of spore? And another type of spore would be-- you could have spores that are all different genotypes from each other and that you have A cap dominant A/B; dominant A, recessive b; recessive a, dominant B; and lowercase a and b. And this is known as tetratype, because there are four types. So how do you get this tetratype? Anyone have an idea? Yeah, Jeremy?
AUDIENCE: You're crossing over. So one of A and B would switch in one of the [INAUDIBLE].
ADAM MARTIN: Where would the crossing over happen?
AUDIENCE: Between the two [INAUDIBLE] one [INAUDIBLE].
ADAM MARTIN: Between the allele and what?
AUDIENCE: Sorry?
ADAM MARTIN: The crossing over would occur between the gene--
AUDIENCE: Oh, and the centromere.
ADAM MARTIN: And the centromere, exactly. Jeremy is exactly right. So Jeremy said that in order to get a tetratype, you have to have a recombination event, but this time, not between two genes, but between a gene and the centromere. So at least one of the genes has to be unlinked to the centromere. And in that case, now you get a meiotic event that gives rise to four spores.
And there are four different ways to get this. So if you have two genes unlinked and at least one is unlinked to the centromere, then you get a pattern where you have a 1 to 1 to 4 ratio between all these different events. So you have a 1 to 1 to 4 ratio between parental ditype, non-parental ditype, and tetratype.
And we can see this in yeast. If you have two genes that are linked to the centromere, you only get parental ditypes and non-parental ditypes, where virtually everything else gives rise to tetratypes, except if they're linked. What happens if the two genes are linked to each other, irregardless of the centromere? If you have two genes that are linked, what's going to be-- what are your progeny going to look like?
AUDIENCE: Parentals.
ADAM MARTIN: You're only going to get the parentals, or you're going to get a lot of parentals. Javier is exactly right, right? If the two genes are linked, the parental ditypes are going to be much greater than any of the other classes. Now, this might seem esoteric, but I like the idea that you can have linkage between a gene and something that's just the place on the chromosome that's getting physically pulled. It all makes it much more physical, which I think is nice to think about.
All right, we're almost done. I just have-- yes, Natalie?
AUDIENCE: Can you go over what the PD [INAUDIBLE]?
ADAM MARTIN: Yes. So PD is parental ditype. So this is the parental ditype. It's a class of product here where you get four spores that are each of these genotypes, OK? So each of these 1, 2, 3, and 4 would represent one of these cells from a single meiotic event. Does that make sense, Natalie? Does everyone see what I did there? So these 1 2 and 3 are the spores of the meiotic event right here.