Flash and JavaScript are required for this feature.
Download the video from Internet Archive.
Description
Professor Martin discusses the key features of cells, from the relatively simple organization of prokaryotic cells to the more complex organization of eukaryotic cells. He then covers cell compartments, transport, mitochondria, and the cytoskeleton.
Instructor: Adam Martin
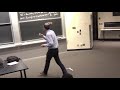
Lecture 11:Cells, The Simpl...
ADAM MARTIN: So, last semester, my grandfather passed away, and I was responsible for explaining to my two sons how a funeral works. So I'm a professor, right? I pride myself on being able to explain things clearly. So I went to tell my five-year-old son sort of what's going on during the funeral. I told him, your papa's body is up in that wooden box up there. We're going to celebrate him right now. We're going to go to the cemetery, and then we're going to bury him.
And you know, I'm a professor. I thought I nailed it, OK? Except my son was showing this looking look of concern in his eyes. And he looked at me. He was like, what about his head? So my plea for this semester is please let me know when I've forgotten the part about the head, OK?
You know, you guys are listening to me. I might have forgotten something that, to me, seems kind of obvious, but that, for you, it would help to know to understand the material. So today, we're going to start with cells. And so here, you can see this is one of my favorite movies. This is a neutrophil cell here that's migrating. You can see it's chasing after this smaller cell which is a bacteria cell. And around this neutrophil, what you're seeing here are these red blood cells, which aren't doing very much of anything.
So the point I'm making with this video is that cells have a huge amount of diversity. So cells have diversity. As you can see from the video, there's a diversity in size. There's diversity in shape and also diversity in behavior.
OK, so we're going to unpack this a little bit. And I just want to start by just pointing out that until now in the semester, we've dealt mainly with very small things, such as atoms, small molecules, lipids, and proteins. And the size of these structures, they're on the nanometer scale. Now, cells are a unit up in size. Cells span several orders of magnitude in size.
So bacterial cells are on the small side. They're on the 1 micron to 10 micron size. So you can see that here. Most bacteria is about 1 micron to 10 micron. But our cells span from tens of microns to hundreds of microns, and even larger than that, because the human egg is on the order of a millimeter in size. This is not a human egg, but this is a frog egg. You can see often the egg cells are the biggest of all cells. The biggest cell is an ostrich egg. It's about 15 centimeters, I believe.
So cells span a huge, wide range of sizes. I'm going to start with the simplest, which is a bacteria cell. And what I want to point out about this cell is its simplicity. So here, you can see this is an electron micrograph of bacterial cell here. And this is a cartoon just illustrating some of the key features. You have a plasma membrane and a cell wall. The cell wall here is in sort of-- the periplasmic space is in green. And this encapsulates the cytoplasm.
And the only other real structure you can see, in this case, is this nucleoid structure in the middle. And what the nucleoid is, is it's just the chromosome of the bacterium. And I want to point out that later on in the course, Professor Imperiali is going to come back and tell you about antibiotics and how bacteria can develop antibiotic resistance, which is of critical importance for biology and medicine.
So our cells are more complicated than this. And that's because if you look at this EM here, you can see that eukaryotic cells-- and we are eukaryotes-- have membrane-bound compartments. There's a nucleus here that houses our nuclear DNA. And also, there's a series of membrane compartments that span the cytoplasm.
Now, our cells, even in a single organism, such as us, our cells have incredible diversity and specialization. So there's diversity within a single organism. There is specialization. So as we develop from a single cell, our cells acquire properties that allow them to carry out specific functions in our bodies. And an extreme example of this is shown up here.
These are pictures or drawings of neurons from Ramon y Cajal, and you can see how this looks nothing like the cartoon picture of a cell I just showed you. These cells have highly dendritic sort of arrays of protrusions. And these cells have evolved such that they are very good at sort of transmitting information in the body, right? An extreme example of a nerve is a sciatic nerve, which extends from the base of your spinal cord all the way down into your foot. So it's about a meter long. That's an extreme specialization for a cell.
So these cells are specialized, but what's important to note is that within a single organism, the genomic DNA of a cell is more or less the same. So genomic DNA is the same, with some exceptions, some of which we'll get to in the course. The genomic DNA is the same. What's different is the genes that are being expressed in these cells and the proteins that they encode that give these cells different functions. So what's different and what allows cells to acquire these different functionalities is this different gene expression.
OK, so that's the overview. Now I want to talk about compartments. And if we go back to this cartoon that I showed you from your-- oh, I just wanted to point out that right now, at MIT and the Broad, there is a project that's ongoing to really define all the cell types that are present in humans. And this is known as the Human Cell Atlas. And I just want you to take a minute to think about if you wanted to define different cell types, what would you look at to classify these cell types? Anyone have an idea? Yes? What's your name?
AUDIENCE: Rachel.
ADAM MARTIN: Rachel.
AUDIENCE: Cell function.
ADAM MARTIN: What's that?
AUDIENCE: Function.
ADAM MARTIN: You could look at function. That might be a little subjective in how to interpret. What defines the function of the cell?
AUDIENCE: I'm thinking like what it does and how it works with other cells.
ADAM MARTIN: But is there something, I guess, within that cell that would define what its function is? Yeah? What's your name?
AUDIENCE: Samantar
ADAM MARTIN: Samantar?
AUDIENCE: Samantar
ADAM MARTIN: Samantac All right, yeah?
AUDIENCE: Gene expression data.
ADAM MARTIN: Gene expression, right? What genes are these cells expressing? So what they're doing is they're isolating single cells from tissues. And then they're looking at gene expression. So which type of molecule here would be the molecule you'd want to look at if you want to look at gene expression? Not Miles. Malik.
AUDIENCE: You look at mRNA.
ADAM MARTIN: mRNA, exactly. Malik is exactly right. So you look at the mRNA, right? Because if there's mRNA, that means that gene was expressed, and it's possibly encoding the translation of a protein. So that's what they're doing. They're isolating single cells and doing a massive single cell RNA seek project. And that's helping to identify new cell types in the human body.
OK, coming back to compartments. So I showed you this picture. You can see there are lots of membrane-bound compartments in the cell. You can see the lines in this cartoon represent lipid bilayers. And so these are compartments that are completely encapsulated by a lipid bilayer. And I'm going to remind you of something that Professor Imperiali told you about already. And the reason I'm saying it again is because it is so freaking important, OK?
So if we consider a lipid bilayer where the circles are the polar head groups of the lipids and the squiggly lines are the fatty acid chains-- I'm not going to draw all the fatty acid chains. So this is a lipid bilayer. And these head groups, are they hydrophilic or hydrophobic? Yes? What's your name?
AUDIENCE: Stephen.
ADAM MARTIN: Stephen.
AUDIENCE: They're hydrophilic.
ADAM MARTIN: They're hydrophilic. They like water, right? The water is on the different faces here. These are hydrophilic. How about this central region here? Yes? Name?
AUDIENCE: Ory.
ADAM MARTIN: Ory.
AUDIENCE: Hydrophobic.
ADAM MARTIN: Hydrophobic, good, right? That's excellent. So these are hydrophobic. Hydrophobic. So what that means is you have this hydrophobic layer that is surrounding each of these compartments, and that's going to serve as a barrier such that water and things dissolved in water cannot pass through this barrier unless something allows it to. So each of these compartments are membrane bound, have properties in the lumen that's in the interior of the compartment that's going to be different from that of the cytoplasm. And the cell, the interior of the cytoplasm, is going to be different from that of the extracellular space.
So let me just give you some examples of how things are different inside and outside the cell and also inside and outside these compartments. So if we consider the plasma versus the cytoplasm, we can consider the concentration of various ions. And I want to get the concentrations right. Let's consider sodium, which is a monovalent cation, potassium, and calcium. I'm just going to use these as illustrations.
So sodium is concentrated outside the cell, about 150 millimolar, and is less concentrated in the cytoplasm. So there's a huge difference in sodium between the outside and the inside of the cell. Potassium-- now, you might think potassium would be a lot like sodium. It's a monovalent cation. It's a similar size. But it actually shows the flip distribution. So it's four millimolar in the exoplasm and 140 millimolar in the cytoplasm, OK?
So there appears to be some selectivity here, right? The cell is concentrating certain things inside the cytoplasm, and it's excluding others. So there's selectivity, even between closely related atoms here. OK, calcium is two millimolar in the exoplasm and around 10 to the negative fifth millimolar in the cytoplasm.
So because there are these huge gradients in the concentration of these ions leads you to expect that this is a non-equilibrium state. Because if it were equilibrium, these ions would go in and out, and they'd equilibrate such that the same concentration would be on the inside as the outside. And so there's selectivity. And also, there's non-equilibrium, which suggests that energy is required to maintain this asymmetry.
I want to point out it's not just the concentrations of various molecules that are different between the inside and the outside, but the plasma membrane can also have a voltage across it. So this is exoplasm. This is cytoplasm. And this membrane can hold a voltage.
And this is going to become incredibly important when we talk about neurons, because neurons use changes in this voltage to transmit signals across their length and also to transmit signals at the synapse. And we'll talk about that later in the course. So this is known as membrane potential, this voltage difference.
I also, again, want to point out this endomembrane system in here, where the gray regions here are sort of compartments that will communicate with each other. And so there's this whole internal structure of endomembrane system which is compartmentalized from the cytoplasm, OK? And so now I want to talk about how do things get in and out of this structure. So I think we're up to three, getting in and out.
So cells-- and this is very important in cell communication, right? For cells to communicate, cells have to send things, like signals, to other cells. And also, cells can take in stuff and sort of receive things from other cells. So how is it that this happens? Well, if we consider the plasma membrane of a cell-- this is a lipid bilayer, PM. I abbreviate Plasma Membrane, PM. So that's a lipid bilayer.
And let's say that there's some type of sort of molecule that's on the outside of the cell. So, in this case, this would be exoplasm out here. This would be inside the cytoplasm here. Let's say this cell wanted to sort of take up this pink structure into the cell. How would it do that?
Well, this pink structure, because it's hydrophilic, cannot pass through the lipid bilayer. So the cell has to use another strategy to take this up into the cell. Let's see. I'll use a little blue here. So let's say the region of the membrane right here is in blue. Then, what can happen is this blue structure can invaginate, and it can take up this pink molecule.
So the plasma membrane can invaginate. The plasma membrane can invaginate, and if it has this pink molecule, then if there is a cision event here, you've now gone from having your pink molecule on the outside of the cell to having the pink molecule in this vesicle or circular structure on the inside of the cell. So here now we have this. We have our vesicle in blue. And now the cell has picked up this pink molecule, such like this.
So this process of sort of taking material from the outside and sort of bringing it into the inside of the cell is known as endocytosis, which is the process of taking something from the outside and bringing it in the cell, this is also a way that viruses can get in your cells, which is a more nefarious way that this system is used. All right, I need a volunteer. Yes. Ory, come on down.
Don't worry. This'll be simple. I just need you to put pressure on my head right here. So you see the tassel. Make sure they can see the tassel. You see this tassel? This tassel is that pink molecule, is right now on the outside, right? But I'm going to endocytose it by going like that, right? And I also got a hand. That's great.
So you see, that's basically endocytosis. I just endocytosed my tassel. All right, you can go up. We're all set, yeah. I can do the next one. OK, so the opposite of this process-- let me get another color here. You can also have vesicles that are on the inside that then fuse with the plasma membrane and release their contents to the exterior. And this is called exocytosis.
So exocytosis is something that's starting out inside one of these vesicles and then goes out, OK? So this is not exactly reversible, because there are different protein machineries that mediate either endocytosis or exocytosis. So now I'm going to exocytose my tassel. There we go. So I just exocytosed it, and now my molecules is again facing the outside world. So this is a way for cells to sort of taken things and also to secrete molecules, like signaling molecules, into the extracellular space.
So now, we're moving on, and now we're going to talk about compartments within compartments. So we're on four-- compartments within compartments. And you're going to see how this relates to endocytosis in just a minute. So compartments within compartments. So the example I'm going to use here is an organelle that is present in us, in animal cells, which is the mitochondria.
And you all know that the mitochondria is the powerhouse of the cell. Whenever I say mitochondria is the powerhouse of the cells, I have to do 10 push-ups, because it's so cliche. I mean, my five-year-old knows that mitochondria is the powerhouse of the cell. It's a gross oversimplification, OK? Mitochondria are way more interesting than that, and I'll show you in just a minute.
All right, I'll draw mitochondria, the same mitochondria that my kids draw me. So here's a mitochondria. I'm drawing first the outer membrane. So there's an outer membrane to the mitochondria. And this organelle also has an inner membrane. So this is the inner membrane.
The inside here is called the matrix. I'll draw some DNA molecules there. So this is known as the mitochondrial DNA, which I'll mention in just a minute. So this is the mitochondria. Where could such an organelle come from, evolutionarily speaking? You know, one problem that eukaryotic cells have is they cannot make mitochondria de novo. The way that mitochondria is passed on is it's replicated during cell division, and it's passed on from one cell to the next.
And you guys all got your mitochondrial DNA and your mitochondria, at least initially, from your mother. So this is not an organelle that can be synthesized de novo. And the fact that this is the case has led to a theory known as the endosymbiont theory, or hypothesis, which basically states that there was an ancestral eukaryotic cell. And the way that organelles such as mitochondria and plastids were derived is from engulfing bacterial cells that were either capable of oxidative phosphorylation, in the case of mitochondria, or were capable of photosynthesis, in the case of chloroplasts.
And so this engulfment is much like this endocytic process that I just talked to you. It's not really endocytosis, because these bacteria are much better than an endocytic vesicle. So it's more of like a macro sort of pinocytosis or something like that, OK?
So something you may have seen in the news lately-- so some of the evidence for this endosymbiont theory is that mitochondria and plastids have their own DNA, OK? So these organelles have retained DNA. The genes in the DNA encode for proteins, that function in the mitochondria. It also includes ribosomal RNAs and transfer RNAs that are required for protein synthesis within the mitochondria. But a lot of the genes that are required for a functioning organism have now been exported to our nuclear DNA, and those genes are made and proteins are produced, and then they're imported into the mitochondria. But the mitochondria has retained a number of genes, and it's encoded in the mitochondrial DNA.
Another reason to think that this could be from a symbiotic relationship, this ancient, between eukaryotic and prokaryotic cells is that these organelles divide by fission similar to how bacteria divide. So they divide by fission. So I'll show you a real mitochondria and a mitochondria undergoing fission in just a minute. I just want to point out that in the news recently, there's been talk of three-parent babies. And I just want to explain to you what that is.
So eggs that are-- I shouldn't say embryos, but eggs that have the nuclear DNA from two parents can then be given mitochondrial DNA from another parent. So these three-parent babies are essentially babies that have nuclear DNA from two parents but mitochondrial DNA from a third parent. So here's just an article in the NewScientist reporting that this is imminent, and now it's been done.
Now, why would you might want to do that? Anyone know why someone would want to do this? Yes?
AUDIENCE: There might be a defect in the mitochondrial DNA of both parents.
ADAM MARTIN: Yeah. So Stephen, right?
AUDIENCE: Yes.
ADAM MARTIN: Stephen's exactly right, right? There are diseases that are so associated with faulty mitochondrial DNA. And if you're a mother and you have the mutations that cause this disease, this would be a way for you to have a child without passing on the disease to your child. And so this is something that is still controversial, but that's why people are exploring the opportunity for making these so-called three-parent babies.
Again, I've a pet peeve with textbook pictures of mitochondria. Here's your textbook picture. Everything's labeled nicely. This is what mitochondria look like in real life. Mitochondria, like the endoplasmic reticulum, actually, form these tubular networks that essentially span the entire cell. So it's convenient for us to depict mitochondria like this in textbook, but mitochondria are way more interesting than that. They're dynamic organelles.
And I also want to make the point-- we kind of talk about these organelles like they behave as these separate entities, but in fact, they interact with each other. And there's lots of interesting biology behind that. So I'm going to show you one movie that's from work done by Gia Voeltz, and this Friedman person is the first author. She just gave a talk here at MIT and showed some beautiful movies.
All right, focus on this movie here. The ER is labeled in green, and the mitochondria is in red. And what you see is there is this mitochondria tubule, and it's crossed by the ER right here. Now, focus on this when the movie plays. You're going to see that the mitochondria undergoes fission right at these points, where the ER and the mitochondria intersect. So that illustrates-- and now they've mechanistically dissected what sort of makes the mitochondria undergo fission at these crossing sites.
But it really illustrates the real sort of complexity and dynamics that are present in a cell, which you might not be getting from your book. Oh, I did want to mention that-- so there's a chapter in your book-- I think it's Key Concept 5.3-- where you can read about all the organelles. You should read that and know roughly what the organelles do. I mean, I could lecture about that, but it would just be so boring that I can't do it. I'd have to do a ton of push-ups.
So we have to sort of choose what we lecture about. So I would just suggest you read that part in the textbook. If you have questions, come talk to me. And just be familiar with what your organelles are doing. Yes, Ory?
AUDIENCE: What was the chapter?
ADAM MARTIN: It's 5.3.
AUDIENCE: Is it like a chapter?
ADAM MARTIN: It's Key Concept 5.3. It's probably listed in the assignments, right?
AUDIENCE: It's a section in the chapter.
ADAM MARTIN: Yeah.
AUDIENCE: It's only a little bit. It's not going to be long.
ADAM MARTIN: All right. I have one last part, which is to prepare you for Friday's lecture. In Friday's lecture, we're going to start talking about genetics. And before we talk about genetics, we're going to talk about something that is-- we're going to lay the groundwork, essentially, for genetics by talking about how cells divide.
So I think one of the most miraculous things that cells do is that they can undergo this trick where they replicate themselves and split it into two daughter cells. And you're seeing here, there are chromosomes in the middle here. They're going to line up along the metaphase plate. And now they're going to get pulled to separate sides. They're going to wiggle back and forth first.
Then, in just a minute, they're going to get segregated. They'll go eventually. There they go. So you see-- and then the cell is going to pinch at the equator and divide in two, OK? So now I'm at this last part, the cytoskeleton, because the cytoskeleton is the answer to part of how these cells divide.
And before I present the cytoskeleton, I just want to briefly mention chromosomes and what they look like. So the chromosomes are your nuclear DNA. They're linear pieces of DNA, as opposed to the mitochondria, which has circular DNA. And again, the fact that mitochondria have circular DNA is analogous with sort of bacterial chromosomes, where bacteria have circular DNA. But our chromosomes are linear pieces of DNA.
And you guys have probably all seen chromosome spreads that look like this. So this would be a chromosome that's replicated. There are two copies, one and two. So this is a replicated and condensed chromosome, right? Initially, chromosomes are just like bowls of spaghetti. Everything's mixed together. But during mitosis, the chromosomes condense, and then they sort of resolve from each other, such that you can see the arms of the chromosome. And you can see where the chromosomes are coupled to each other at this structure here, which is known as the centromere.
And at this centromere, a protein complex assembles. I'll just draw it like this. This protein complex is called the kinetochore. The kinetochore is a complex of hundreds of proteins that assemble into this large platform that sits on the centromere. And the kinetochore is able to attach to the cell's cytoskeleton, specifically microtubules. So this attaches to microtubules. And I will tell you what microtubules are right now.
So microtubules are a component of the cell's cytoskeleton. So the cell's cytoskeleton is a network of filamentous rods that are present in the cell. And the term cytoskeleton kind of makes it sound boring, I think, because it makes it sound static. But the cytoskeleton is anything but static. It's actually a dynamic machine in the cell. And these machines that are assembled from these fibers are able to generate force.
So these microtubules and the cytoskeleton, they generate force. You can think of them as motors or machines. So there are machines that generate force. And I'll just illustrate this with a couple of videos and slides. So, again, this is not a stable structure, but very dynamic. So this is going to be a movie where, in green here, microtubules are labeled, and the red label's the nucleus.
And these cells in this fly embryo are going to undergo cycles of nuclear division. And so you're going to see the microtubules assemble, disassemble, assemble, disassemble. So you see how dynamic this process is. The cell is able to assemble this force-generating apparatus, which is known as the mitotic spindle, each and every cell division. So this structure, or machine, is critical to physically segregate the chromosomes to opposite poles of a cell.
So now I'm going to tell you about the microtubules themselves. So microtubules, as the name implies, are sort of tube-like polymers. So these microtubules are biopolymers, which means that cells are expressing sort of genes that encode for proteins that form a subunit that can then self-assemble into a larger rod-like structure. And you can see one of these rod-like structures here.
They essentially look like straws. They're about 25 nanometers in diameter. And I'm going to show you this video showing you microtubules both disassembling at first and then assembling. So that's a dissembling microtubule. But they also assemble to form these longer rod-like structures. So these biopolymers are dynamic, and they both assemble but also disassemble. And both the assembly and the disassembly can generate force.
Microtubules can push if they polymerize into something. They push it, right? Just like you're kind of poking someone with your finger. Now, they also disassemble, and when they shrink and disassemble, they can actually pull. And I'll show you an example of that right here. So this is an example that's reconstituted, meaning they're just purified proteins here. There's no cell.
And what this is, is a bead. And you see the dark stripe here is a microtubule. You can see it growing. There's the end right there. That microtubule's going to grow. It's going to grow out to about here. And then at the end of the movie, it's going to stop growing, and the microtubule's going to shrink back. And you're going to see that when the microtubule shrinks back, it's going to actually pull this large bead and pull it towards the left side of the slide.
Here it goes. It depolymerizes and pulls. You see it? So these microtubules can generate a pulling force that's strong enough to pull this glass bead. And also, it's strong enough to pull an entire chromosome. It's actually much stronger-- it generates much more force than it requires to pull a chromosome. So it seems like there's some robustness in the system so that it's generating a pull that's much stronger than needed to actually drag a chromosome through viscus media.
So during mitosis, the way this system is set up is there's what's known as a bipolar spindle. I'll just write down that. We'll come back to it in Friday's lecture. There's a bipolar spindle. And the bipolar spindle is made up of a number of microtubules. So here, you can see there are two poles, here and here. And in the middle, along the sort of equator of the cell, the chromosomes will line up. There are just two chromosomes here.
And you can see how microtubules reach out from the pole and attach to both sides of that chromosome. And you can imagine that when these microtubules are told to shrink and disassemble, if they're able to remain attached to that kinetochore, which they are, when they shrink, they're going to pull the two copies of the chromosome away from each other, OK? And so this is the basic machinery that allows for chromosomes to be segregated in cells.
OK, that's it for today. Any questions? All right, terrific. Good luck on your exam on Wednesday. It's in here.