Flash and JavaScript are required for this feature.
Download the video from Internet Archive.
Description
In this lecture on model organisms, Professor Martin discusses how to go from a phenotype of interest (such as appearance or behavior) to identifying the genes and mechanisms that are important for that trait.
Instructor: Adam Martin
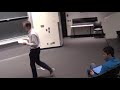
Lecture 15: Genetics 4—Th...
ADAM MARTIN: All right, so today, I'm trying something different this semester. I wanted to tell you guys about one way that you can discover things in biology. And this is going to fall in the genetics cluster of lectures. I want to show you how you can go from being interested in some property of an organism, or even its behavior-- how would you go from there to identifying genes and mechanisms that are responsible for that type of behavior or appearance?
OK, so today, we're going to go from some type of phenotype, or process that you're interested in, such as maybe the appearance of an organism. But we're even going to go more abstract than that because at the end, I'm going to tell you if you're interested in behavior, you can try to figure out the genes and mechanisms that are involved in determining the behavior of an organism. So the question is, how do you go from something you're interested in learning about an organism to actually identifying genes and mechanisms that are important for that?
And on my title slide here, I have three fruit fly mutant phenotypes that you can see, and each of these mutants defined genes that were subsequently found to be present-- or homologous genes were present in humans and were shown to play important roles in human biology. So later on in the lecture, hall kind explain what each of these phenotypes is. But first, I want to just highlight the importance of model organisms and their use in biology.
You've been seeing them already. I've talked a bit about flies, we talked about Mendel's pea plants. I just now have a compendium of model organisms that I'm going to throw up to tell you about. So we've talked about bacteria and the importance of bacteria in elucidating the flow of information from DNA to RNA to protein.
Bacteria we're also extremely important for elucidating the initial mechanisms of gene regulation. I mentioned yeast a little bit in the last lecture when I mentioned tetrad analysis, but we'll talk about yeast a lot more later, when we start talking about the regulation of cell division and the cell cycle, because yeast played a pivotal role in identifying the gene that's critical for this decision of a cell whether or not to divide.
Also, one terrific model organism in plants is a Arabidopsis Thaliana. And Arabidopsis has also played an important role in elucidating mechanisms of development, but also, in making advances in scientific research that relate to agriculture, such as disease resistance and pathogen interactions.
So the two heroes of today's lecture will be the roundworm, Caenorhabditis elegans, and the fruit fly, Drosophila melanogaster. But before getting into study is in those organisms, I wanted to highlight a couple important vertebrate-model organisms-- the zebrafish and the mouse. And so you can see the mouse is our lab mascot, but it's also an important genetic model. In particular, there are many models in mice that mimic cancer. And so these have been useful for elucidating mechanisms of cancer.
So it's really unethical for us to do a lot of different types of experiments on humans, but I will mention that there are human cell lines, such as the HeLa cell line, and these also play an important role in biology research. But these cell lines are taken out of context. They're not functioning in the context of an entire organism. So human cell lines are important, but you have to understand that they're sort of an in-vitro system, that they're out of the context of a functioning organism.
OK, so why is it that we research these model organisms? So there are some practical reasons. Most of them are fairly small, and they're easy to house large numbers of them in a lab. They're often cheap to house in the lab and work with. Also, they develop fast.
And especially when we consider genetics, the rate-limiting step in genetics research is the time it takes from the conception of an organism to the time that that organism can reproduce sexually. So these model organisms-- most of them reproduce very quickly, and so that accelerates the pace of research.
But maybe most importantly is the fact that we are related to each of these model organisms through evolution because we all arose from a common ancestor. And just to highlight this example, using the fruit fly, the fruit fly has 17,000 genes when compared to our 20,000 genes, so we have roughly the same order of magnitude number of genes as the fruit fly.
And so let's think about important genes. Let's think about just genes that are associated with human diseases. If we just consider human-disease causing genes, 75% of the human-disease causing genes have a homologous gene in the fruit fly. So we're similar to these model organisms, such as the fruit fly, in particular, genes that are important for understanding human disease.
So I came across this quote when preparing for the lecture, and I liked it. It's from John Rinn, who is a contemporary of ours. And John Rinn said, "Genetic approaches are as fundamental to biology as math is to physics." And I think this is an apt quote because both genetics and math themselves are scientific disciplines, but they can be leveraged to learn things about biology and physics respectively. So genetics really plays a fundamental role in biology and the discovery of new biological mechanisms.
OK, so now, I want to just briefly take you through, how is it that we discover things using genetics? And I'm going to take you through a type of approach that's called a "forward genetic screen." And I'll get to the orchestra in just a minute, but I briefly just want to define what a forward genetic screen is.
So a forward genetic screen is type of approach you would do if you don't know the genes that are involved in a specific process, but you want to identify them. So in a forward genetic screen, you don't know the genes and mechanisms involved. So you don't know what these genes are. You might not even have a genome sequence of the organism.
You don't need a genome sequence in order to do a forward genetic screen because you don't know the genes, but you're interested in a particular aspect of development, or of organismal function, and you want to identify the genes that are important for that. So when starting a forward genetic screen, you have to, then, infer what a possible phenotype would look like if you broke genes that were involved in that process.
So the mantra of geneticists is that we are going to break genes and then look at the result and see if it gives the phenotype we're interested in. So in a forward genetic screen, you're looking for a phenotype that you would expect if you affected a certain process, if you disrupt a process.
So think about this orchestra up here. Let's say you are interested in what regulates each of these sections in the orchestra. What is the master regulator of this orchestra, if you will?
And so conceptually, what a genetic screen would involve is taking hundreds, maybe thousands, of orchestras like this one, and just shooting an individual in this orchestra, and removing them from the orchestra. And so let's say you remove-- let's say you remove this guy right here, then maybe nothing really happens because they are, like, 30 or 40 other violinists, and that's not the major control circuit. So then you would infer that this gene is not important for the regulation of the orchestra.
But let's say you took out Bernstein, and then you listen to the orchestra and find that the sections are uncoordinated, the bassists start doing crazy things on their own. So then the logic of Drosophila genetics, you would then name that gene uncoordinated and infer that that gene has some important role in coordinating the different sections of the orchestra.
So the goal in genetics is to identify a mutation that alters a gene function that gives you a phenotype that you're interested in. And rather than taking a gun and shooting members of the orchestra, in genetics, you try to identify mutations. So you try to induce mutations.
So we're looking for mutations. And these mutations could be spontaneous mutations, meaning you didn't do anything to induce it, but they just appear as a variant in the population. And so we've talked about Thomas Hunt Morgan and the white mutant, and the white gene.
So the white mutant was a spontaneous mutation. Morgan's lab didn't do anything special. They just looked at lots of flies, and over the decades, they identified this one special male fly that they could work on.
But nowadays, researchers have a way to accelerate this process. And so we can induce mutations. In the way we can induce mutations is by using some type of mutagen.
So for example, you could have some sort of chemical mutagen that increases the error rate in DNA replication, or you could use radiation to induce DNA damage, and that essentially accelerates the frequency of mutations that occur in the genome of an organism. And so the process of mutagenizing an organism isn't specific to genes. You're just inducing random mutations across the genome of the individual.
Let's say this piece I'm drawing here is part of a chromosome, and these boxes are genes. You're inducing random mutations, and maybe one mutation hits this gene. So that's a mutation that might affect the function of an organism. You might have other mutations that are outside genes and may have no effect, or you could have a different kind of mutation which isn't in the coding region of the gene, but maybe affects the regulation of this blue gene over here.
So this is a random process. If you're feeding an organism some chemical mutagen, you're inducing random mutations in different places, and you don't know which are the ones that you want until you look at their phenotypes and try to find the needle in the haystack. So let me show you some examples.
So let's say we were interested in our body pattern. We all have a body. Our head is in our head position, our ass is in our ass position, we have arms that are in a right position, our legs are in the right position. Let's say we're interested in figuring out what genes were responsible for that body pattern. What kind of a mutant might you look for? Anyone? Rachel.
AUDIENCE: [INAUDIBLE]
ADAM MARTIN: What's that? A mutant with his body parts in the wrong place. Maybe you have like a leg where your arm should be coming out, or maybe you have two heads, or something like that. So you look for some sort of defect in the pattern formation.
And so this would be, obviously, unethical to do in humans, but in model organisms, we can actually find these types of mutations. I'm just going to highlight a couple mutants. This one's an obvious one.
So this fly has two wings. You can see them folded over each other right here. But there's a specific class of mutant that was called "wingless"-- where the flies have fewer than two wings. This particular fly has only one wing.
And this wingless mutant defined a gene that became known as wingless, and it's a gene that has a homologous gene in humans. And this particular gene defines an entire signaling pathway, which-- whoops, sorry-- which is important in stem-cell biology and is also over activated in cancer.
But obviously, we don't have wings, so this gene didn't get discovered in humans. It was discovered in flies, and then only later on, it was inferred-- or it was discovered that there is a related gene in humans. OK, so that's one example.
One of the other phenotypes I showed you is called "notch." Normally, a fly wing has a nice, smooth margin. But notch mutants have wings that have this chunk taken out of them at the end. So again, the gene became known as "notch" because of this fly phenotype. But again, there's a human notch, and human notch, again, is involved in human diseases, such as cancer. OK, so that's two examples.
But now, I want to talk to you about how one might find the needle in the haystack. How can you have a concerted effort to identify genes that have that function in a given process? I'm going to tell you about work done by Eric Wieschaus and Christiane Nusslein-Volhard because they did one of the more famous genetic screens that had been done, and they won the Nobel Prize for their results in 1995. So I mean I take you through this classic genetic screen.
In this screen, they're going to induce mutations. So I'm going to take a parental generation. And this screen was done using fruit flies.
And they took male fruit flies and treated the males with a mutagen to induce mutations in the gametes these male flies that would then be passed to subsequent generations. And they mated the mutagenized males to females, and then they went on to look at-- to isolate individual F1 progeny. So we're going to look at individual F1 progeny in this generation.
So in the F1 progeny have the potential to get one mutagenized chromosome from their father and will get a normal chromosome from the mother. So I'll just drawn this like this, where independent mutations are going to be different colors. So that has maybe a mutation on one of its chromosomes.
Another fly, because this is random, might have a different mutation on the same or a different chromosome. I'll draw a couple more. And maybe one of these flies doesn't have a mutation that's been induced. And then I'll draw just one independent mutation right there. So again, this is random. So to see random mutations, you have to take individual flies.
Now, are you're going to see a phenotype in this F1 generation? So Miles-- is it the Malik or Miles? Sorry.
AUDIENCE: Miles.
ADAM MARTIN: Miles. Good. OK.
AUDIENCE: Most likely not because any mutation [INAUDIBLE] spontaneous would be overshadowed by the direct gene, the female.
ADAM MARTIN: Exactly. So what Miles just said is that-- basically, he said that these mutations if their loss-of-function mutations are going to be recessive-- because they're going to be overshadowed by a normal functioning gene on the other chromosome. So if you're looking for a loss-of-function mutation, that's likely going to be recessive, and you would not see it in the F1 generation.
So in order to look for organisms that have incorrect body patterning, you need to get a situation where each of the mutated chromosomes is homozygous recessive. And to do that, you have to do more crosses.
So what the researchers did was to take independent individual organisms, and cross them all, again, to non-mutated flies. And remember, flies don't self-cross. So they need a male and a female that has the same mutated chromosome mating to each other in order to see a recessive phenotype.
So because you only have an individual fly for each of these, you need to get more than one fly with the same mutation. So they mate to a normal fly. But now, you can get an F2-- multiple F2 males and females that will have the same mutated chromosome.
So here, let's draw these. They're still heterozygous. At this point. But at this point, you just generated multiple organisms, male and female, that have the same chromosome that arose from, essentially, the same chromosome and the father, or the male gamete and the parental generation. Oh, I did green. OK.
OK, so now we have males and females, both of which are heterozygous. And I'm skipping some of the details of this cross. You'll want to take my word for the fact that they can keep track of which chromosome is which. And I'm not going to tell you how they did that because it's fly genetics, and it's not terribly important that you know it.
So now, you have males and females. And now you can look an F3. And from each of these lines-- each of these lines, you're going to cross siblings to each other. So you're doing a sibling-cross.
What fraction of the progeny for each of these sibling crosses should be homozygous for the mutant chromosome? Yes, Steven.
AUDIENCE: One-fourth.
ADAM MARTIN: One-fourth, exactly right. So Steven's exactly right. A quarter of the progeny should get two copies of the mutated chromosome. So 25% should be homozygous recessive.
And so you can screen this F3 progeny for each of these independent lines, and look for flies at some stage of development that are defective in patterning. And so I'll show you how-- where'd my clicker go? All right, so they're looking for incorrect body pattern.
Yes, your question-- what's your name again?
AUDIENCE: Georgia.
ADAM MARTIN: Georgia, yeah.
AUDIENCE: Would there be some [INAUDIBLE] in the F2 [INAUDIBLE] normal? [INAUDIBLE]
ADAM MARTIN: Yes. So they're able to select for the mutated chromosome, yeah. And I'm skipping over how they did that because it's kind of esoteric. But you're right. Some would be normal. But I'm just telling you they're able to figure out the ones that have the mutant chromosome, and they made sure to keep those in the next generation. Good question, Georgia.
All right, so they can screen F3 for a phenotype. It turns out all of the mutations they're interested in are lethal, so they have to look at the larval stages for ones that have a defect in patterning.
So this is what a Drosophila larvae looks like. And you can see it has a segmental pattern here. This is the head of the larvae, the tail of the larvae. But you see there are these segments that alternate between smooth cuticle and hairy cuticle. They have things called "denticles," which are these hairlike projections, which are essentially, what the maggot uses for traction so it can crawl around. So they're basically just looking at maggots here and looking at the pattern of segments in the maggots.
But what they found is a mutant. And here's a mutant here which just has these hairlike projections all across the cuticle without these intervening regions of naked cuticle. And because there's a lot of hairlike projections here, it reminded the researchers of a hedgehog, and so this mutant became known as "hedgehog." And the hedgehog gene was the founding member of an entire signaling pathway, known as the "hedgehog pathway," that plays important roles in human development and also, human cancer.
So the human gene for a hedgehog, or one of the most important ones in humans, is known as sonic hedgehog. So you see that geneticists have kind of an odd sense of humor. But the sonic-hedgehog gene is important in cancer.
And actually, there are now a number of drugs that are being developed to target the hedgehog pathway. And one was approved back in 2012 for use in treating basal-cell carcinoma. And there's currently another drug that's in phase-II clinical trials for treating some forms of leukemia. So this is a story that goes from identifying this weird fly mutant all the way to clinical trials, developing drugs, whose purpose is to inhibit this signaling pathway, which hedgehog is the signal-- the extracellular ligand for.
OK, so now, I'm going to switch gears and tell you about another story. It's a bit like this one, except this one has an MIT connection. So I'm going to tell you about work done at MIT in the worm Caenorhabditis elegans.
And this work was done in the lab of Robert Horvitz, who is a member of our biology department. And Robert Horvitz, for his work, won the Nobel Prize, along with John Sulston and Sydney Brenner for their work on how cells decide what fates they give rise to. And one thing that the Horvitz Lab has done is to elucidate the mechanisms that determine whether or not a cell lives or dies during development.
So if we think about the development of an organism, we all start from a single cell. And the cell divides into different cells, but different cells in our body give rise to different cell fates. So if we consider just a generic cell division here, you have a parent cell, A. It might divide into cell B and cell C.
And maybe cell B might have a particular fate. It might go on to develop into a neuron, or a muscle cell, whatever. It doesn't matter.
But one fate that Horvitz, Sulston, and Brenner saw is that sometimes, cells-- their fate was to just die. And this was very stereotypical. You get a death. And this was defined as "programmed cell death" because it followed a very stereotypic pattern, where the same cell, each time, would undergo cell death, so it seems like there's a program for it. This also is called "apoptosis."
So what really enabled this work is the biology of C. elegans. And, here, I'm showing you C. elegans development. This is the C. elegans zygote. This cell divides into two cells that are different. One is called AB, one is called P1. And we know exactly what the fates for the descendants of both these cells are based on the work of Sulston, Brenner, and Horvitz.
Now, you get a four-cell stage where AB divides into two cells, and P1 divides into two cells. And again, we know the fates of all these cells.
And what C. elegans researchers have done, because C. elegans is a relatively simple organism-- there are only 947 cells in every individual worm, so there are 947 somatic cells. And this is stereotypic. Every worm has these 947 cells.
How many cells do you have? More than or less than 1,000? You have more than 1,000 cells, right? That makes you much more complicated.
So really, the practical aspect of C. elegans is it has a much simpler composition, and they can track what happens to every single one of these cells. They know when every cell divides and what the daughter cells of that division will turn into. In other words, they know the entire lineage of this animal. So this is a picture of the lineage. That's showing you what happens to every single cell in the development of an adult worm.
And so what particularly interested Robert Horvitz is 131 cells, during the development of this animal, underwent programmed cell death. And this is not random cell death. It's the same cells every time. They know what happens to every single cell in the development of this organism, so they know when there's a division, and one specific cell dies every time in the organism. So it's really a unique scenario, where you can really see what becomes of every cell that is present in the organism.
OK, so now, let's talk a little bit about the death process. So I'll make the cell-death process simple. You start with a live cell. So you have a live cell. That live cell dies such that you then have a dead cell.
And then after the cell dies-- after the cell dies, the remnants of that dead cell are engulfed by neighboring cells, such that you no longer see that cell. So the last step in this process is engulfment.
Now, a key aspect of one of Horvitz's screens is that other researchers had identified a mutation that affected cell death that specifically blocked this engulfment process. And this gene is called ced-1. There's the first cell-death mutant that was identified.
"Ced" stands for "Cell Death abnormal" so C-E-D is short for "ced." So researchers in the C. elegans field started isolating these cell-death-abnormal mutants, where something happened in the cell-death process that was abnormal. And I'll tell you how this was leveraged by the Horvitz lab to identify, basically, a pathway of genes that were involved in cell death.
So this is now a worm. And what you see in this worm are these bubble-like structures that are cells that are dead but haven't been engulfed. So these dead cells, because they're not engulfed, are visible in the adult worm. And so you can basically just look at adult worms, and see whether or not these bubble-like structures are present in a ced- mutant. In a wild-type worm, you don't see this, and so it's harder to see.
But this provided a visual assay to look for mutations that block the cell-death process because if you block the process upstream of ced-1 in an engulfment, you no longer will see these bubble-like structures. And so that is what the Horvitz Lab did.
They started with ced-1 mutant worms. So they're starting with the ced-1 mutants. And they then mutagenized these ced-1 mutants. Sorry-- mutagenized. And so they're essentially, looking for second mutants in this animal that will affect the death process. OK, I'll take you through the logic of this.
So for the remainder of the generations I'm going to tell you about, they're all ced-1 mutant. Ced-1 is homozygous, in this case. And the worms are hermaphrodites, meaning they are both male and female sex organs. And therefore, they can self-fertilize. So this is a self-cross. And so ced-1 remains homozygous mutant throughout all these crosses.
Now similar to the fly screen, they can look for individual-- or you get individual worms here. Let's get some colors so we can compare different chromosomes. All right, so this chromosome might have one mutant, this chromosome could have another mutant, and this chromosome could have another mutant, and maybe this one doesn't have a mutant.
OK, but again, these are heterozygous animals, so if you're looking for a loss-of-function mutant, you would not see the phenotype at this stage. So what was done in this screen is, because these are worms and they're hermaphrodites, they basically allowed each of these worms to self-cross. So now, were talking just about a self-cross.
And because a single worm has just one of these chromosomes, when it undergoes a self-cross, a quarter of the progeny will be homozygous recessive for the mutation. OK, so here, we have the F1 generation. And now, they can just look at the F2 generation, and they're looking for some fraction of the worms resulting from each individual that has a phenotype that looks like a cell-death mutant phenotype.
And so they're essentially, going to screen through the F2 generation, and look for worms that fail to have these bubble-like structures in the adult world. So they're looking for a loss of these refractile structures. And they got one. This is now a double mutant between ced-1 and ced-3, and you see how you no longer have these big bubble-like structures in the worm. So they identified a mutant, and thus, a gene, that's called "ced-3," which basically causes a failure of the cells to undergo cell death.
Now given what I've given what I've shown you, is this necessarily a mutant that's involved in cell death? Can you think of an alternative explanation for why you would lose these sort of bubble-like structures? What else could be happening?
When you do a screen like this, and when you do science in general, you have to think through all the different types of possibilities. Yes, Georgia.
AUDIENCE: [INAUDIBLE]
ADAM MARTIN: Excellent. Georgia said you could have re-mutated ced-1. So one possibility is that you isolated a ced-1 revertant, which means you changed its DNA sequence so that now, the ced-1 gene is functional.
So one scenario here is you could have some type of revertant, or you could have a suppressor mutation. Maybe you have a mutation that bypasses the function of ced-1 such that now, the cells can engulf the dead cell. So you could also have a suppressor. Or the alternative scenario, the one that we want, is that we've affected the cell-death process and identified something that is a bona-fide cell-death mutant.
How would you differentiate between these two? Any ideas? Should this have an extra cell? No. Stevens says no. I agree. There should be no extra cell here because if you restore the phagocytic process, then the cell should die, it should be engulfed, and there shouldn't be an extra cell.
But if this is a bona-fide cell-death mutant, then you should have extra cells. And it turns out the ced-3 mutant blocks all 131 of these cell deaths so that you have 131 extra cells. Because they know the entire cell lineage of this worm and can see whether or not cells are present or not, they can tell if there are extra cells or not. And therefore, that allowed them to infer that they had an actual mutant that was affecting the mechanism that promotes the cell to die.
And this shows that the cell death-- it shows the cell death is an active process. It's not just some random event that's happening. It's an active process that is controlled by genes. So there's an active mechanism involved in the cell death. Any questions about how these screens go in the crosses before I move on?
OK, so I have one more story to tell you about, and this relates to behavior. So now, I want to tell you about behavior. We all behave, some of us better than others.
So how is it we can go from something as abstract as behavior to specific genes and mechanisms that control this? And I'm going to tell you about work that was awarded the Nobel Prize last year. Two of these researchers-- their careers are at Brandeis. And so what they discovered is the mechanism that controls circadian rhythm in organisms-- so circadian rhythm.
So circadian rhythm is a behavior. We are awake during certain parts of the day and are asleep at night. If you're hidden from the light-dark cycle, you continue this cycle for some amount of time. So there's something intrinsic in our system such that we want to exist on this 24-hour wake-sleep cycle. That's why it's a pain in the ass to travel to the other side of the world.
So we want to identify what controls this behavior. What, then, might be a phenotype you might look for? What would happen if you break circadian rhythm? Yeah, Rachel.
AUDIENCE: [INAUDIBLE]
ADAM MARTIN: It wouldn't have a nice, clear wake-sleep cycle. Exactly.
So this screen-- what they did is to look for flies where they didn't have this robust wake-sleep cycle. So you basically look for flies that are awake at night, and identify the mutations that cause this to happen. OK, I'll show you an example of what the data looks like.
So what each of these lines is each tick mark is a measurement of fly activity. So what you see are flies asleep, they're awake, they're asleep, they're awake. And researchers identified mutants that didn't show this 24-hour cycle. So some are just totally arrhythmic. Other mutants alter the period of this cycle so that it's either longer or shorter.
So this is a really elegant way to look for genes that are important for circadian rhythm. And I'll take you through the genetic screen. This was done by Konopka and Benzer. And they did a nice screen. It involves a genetic trick which I'll show you.
There's a type of chromosome called "attached-X" in flies, where two X chromosomes are fused to each other-- so two X chromosomes fused. And if a fly has two X chromosomes, it's female. If it just has one X chromosome, it's male. So that's determines fly gender.
So then these researchers took males and mutagenized them. And they crossed these males to attached X females. And when you cross males to attached X females, you do something clever, which is half of the progeny dies because you need an X chromosome and you can't have three X chromosomes. But your females are all attached XY. So the females actually get their X chromosomes from their mom, which is the opposite of the way it normally works. And males get their X chromosome from dad because the attached X strain has a Y chromosome.
So this is a little bit of a genetic trick. And the fly-- the reason it was done, in this case, is they wanted to mutate the X chromosome and then have the fathers pass on their X chromosome to their sons because there's only one copy of the X chromosome. So if you get a mutation on X, you don't have to homozygous it because there's only one copy of it. So it's a little bit of a genetic trick that, in this case, served the researchers a generation on their screen. OK, so you take this, and then you identify males now that have a mutated X chromosome, and they have only one X chromosomes so you should be able to observe the behavior even here.
But to establish a line of flies that have this mutation, they then took these F1 mutated males, and crossed them, again, to attached X flies. And again, in doing this, all the males from this cross are mutant. So now, you have multiple males, all of which are mutant. So you can start with a single male F1. And by crossing it to this strain here, you get a lot of males now that have the mutant chromosome, and you can look at their behavior to determine whether you've affected circadian rhythm.
Does everyone understand how the attached X works here? It's kind of a little bit of a trick, but flies have these tools that you can use to save your time in the lab.
OK, so these mutants that the Benzer Lab identified had altered period of the sleep-wake cycle, and therefore, the gene was named "period." So this screen identified a gene called "period." This is a gene. And there's a hammer log of the period gene in humans.
And the gene in humans is associated with familial advanced sleep-phase syndrome. So defects in the genes that were identified in Drosophila actually are relevant to human sleep disorders. All right, I'm all set.