Flash and JavaScript are required for this feature.
Download the video from Internet Archive.
Description
In this first of three lectures on visualizing life, Professor Imperiali talks about the applications of luminescence and fluorescence in service to biology. Today's lecture covers their discovery and how they were developed into widely-used tools.
Instructor: Barbara Imperiali
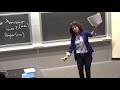
Lecture 27: Visualizing Lif...
PROFESSOR: All right, so fluorescence. You know, I know you hear this from me a lot. But this really is my favorite topic. The applications of luminescence and fluorescence in service to biology are incredibly important.
So what I'm going to try to do in these two lectures is explain to you the difference between fluorophores that we can encode into proteins through genetic engineering and fluorophores that we use that are made by chemists in the lab but then appended to molecules.
So today we'll talk about the nuts and bolts of fluorescence. And then on Wednesday, we'll start to see some of these tools that you've seen images of. We love to wow you with images of fluorescent cells and cells in action.
But I want to step back and actually show you how that all came about. Where do these fluorescent proteins come from? What are we looking for? How much protein engineering was done to make these such an amazingly useful set of molecules, macromolecules to really allow us in real time to study biology?
And there are many, many other applications as well. So we're going to talk about luminescence and fluorescence in general. Luminescence is the general term. And fluorescence is a little bit more specific.
There are different types of luminescence. And you'll get to see some of those varieties of luminescence. I've put a decent amount of our content today on the screen. So we'll go up here and take a look.
So luminescence in general is the emission of light not associated with heat, not like a burning flame which has a lot of light accompanying it. But rather the emission of light in the absence of heat.
And there are different types of luminescence the biologists use intertwined into biological experiments to illuminate life, to understand details of cellular activity. But also as reagents in diagnostics in all kinds of imaging modalities. And through these two-- three lectures, actually, because Professor Martin will give you one that has even more imaging in it-- you'll sort of really get to understand where these pretty magical reagents come from.
So the two types of luminescence that we won't discuss in detail today, first of all chemiluminescence. This is a molecule known as luminol sitting in a little bile. I think I like fluorescence so much because the images are so captivating.
Now, things like luminol, has anyone heard of luminol before? Does anyone-- yeah, do you watch a lot of CSI or-- yeah. So tell everybody what people use luminol for.
AUDIENCE: To pick up on a blood spatter or remnants of blood.
PROFESSOR: Yeah, so when you see these TV shows and there's this beautifully clean motel room and nothing looks like it ever happened there. But people thought there was a murder took place there, you'll notice they come in with these spray bottles. And spray all over the carpets, and the drapes, and the chairs.
And then there's this great moment where they turn off the light and luminol interacts with the heme of blood at an amazingly sensitive level, such that when the lights are turned out, the room's just sort of this battlefield of bright luminescence that indicates that this was a crime scene. So that's the most famous luminol sort of example. So that is what would be called chemiluminescence, the interaction of a chemical with another chemical to give luminescence.
Another pretty useful type of luminescence is bioluminescence. I've done a lot of scuba diving in my life. And there's nothing more exciting than a dive at night where the whole ocean is this sort of inky black.
And sometimes you'll move your arms through the black water at night. And you'll see all these, like, little fireworks. And many, many marine organisms actually undergo bioluminescence. It's a biological reaction that causes luminescence.
And this is a cuttlefish shown here in this image in the corner where they are brightly lit at night. And actually, it's just a whole party there at night in the ocean where all sorts of organisms are signaling to other organisms through bioluminescence and reactions such as luciferase reactions.
So in bioluminescence, a molecule of ATP is generally used and combined with another molecule through the action of an enzyme that ends up kicking out light energy. So those are both important. But what we're going to talk about principally is fluorescence.
This is a more specific term. And you may wonder why I'm putting this in capital letters. The first thing to learn about fluorescence is how to spell fluorescence. So if you look at the word fluorescence and the first word first part of the word looks like flower, you know the stuff you bake your pumpkin pie with, you spelled it wrong. It actually is fluor, F-L-U-O-R-E-S. What are you guys whispering about?
AUDIENCE: [INAUDIBLE].
PROFESSOR: Did I get something else wrong?
AUDIENCE: The E-S.
PROFESSOR: E-S, yeah, well, forget about that part. E-S. There's another C in there. There we go, we snuggled that in.
So the important part is the first part. So make it look like you know what you're talking about and spell fluorescence correctly. I cannot tell you how many papers, scientific papers I read where they spelled fluorescence wrong. It's really hysterical.
It's one of those-- there's two or three amazingly common typos in people's slides. One of them is spelling fluorescence wrong and the other one is spelling complement wrong. Complement as opposed to compliment where you're telling someone they look good. And complement where you're trying to sort of match up things. Anyway, but fluorescence is a key point.
So what is fluorescence? It's the absorption of light energy by a molecule. Now it could be a small, organic molecule. It could be a small part of a fluorescent protein molecule that has a particular structure. But it will absorb light energy at a certain wavelength.
So let's put this into just a cuvette experiment. These are the kinds of little containers that we use for certain types of fluorescence. We could use a plate and a plate reader. But this is quite common. So you shine light on this molecule.
Do you want to nab the doors, the outer doors on both sides? I don't know. Everybody's pretty happy today. Anyway, and the molecule goes. And I'm going to just draw something, you know, with a bunch of double bonds and things.
And the molecule absorbs light and goes to an excited state. So this is the ground state before light. After light you get the molecule in an excited state. So it has absorbed that light energy.
So you've hit it with a wavelength of light. And I'm going to redefine all these terms properly in a moment. Lambda excitation of a particular wavelength. Once that molecule has absorbed light, there's a very transient period until the molecule lets out energy in the form of light. And returns back to its ground state now.
So that the photo physics of fluorescence involves the excitation of a molecule with light of one energy. That light energy is at a particular wavelength so it's called its lambda of excitation. That molecule very, very transiently, it's usually picoseconds or nanoseconds for organic molecules.
It may be a little bit longer for other types of complexes and may stretch to the microsecond or millisecond. But most of what we talk about will be picosecond to nanosecond lifetime. And once it's excited, it just drops its energy back out and goes back to the ground state.
And the most important thing that you want to remember is the wavelength, which is given in nanometers. And the wavelength for emission, which is also in nanometers. This wavelength is higher energy.
Obviously you don't create energy when you shine light on something. You'd be breaking a few fundamental rules if you did. So the light that comes back out is lower energy because there's been rearrangements in the excited state of the molecule. So you can't possibly kick energy out at a higher energy. And so this is a shorter wavelength in nanometers. And this is at a longer wavelength.
So remember, higher energy are shorter wavelength. Lower energy are longer wavelengths. And that is a rule for fluorescence. When you excite a molecule, you'll take it to the excited state. It'll sit and vibrate there a little bit. Then it will kick back energy out at a longer wavelength.
And for the majority of the fluorescence experiments that we do in biology, the wavelengths that you see emission at are in the visible range. Whereas the wavelengths that you might excite your molecule at are often in the UV or a bit longer, ideally, longer.
And these things are going to come up. This isn't the first time that you're going to see them. And it's not the last time you're going to see them. So let's take a look at fluorescent dyes in the electromagnetic spectrum in the next couple of slides.
So what you see here, you see a bunch of little eppendorf vials with fluorescent molecules that emit light at all different wavelengths. These would be down at the ultraviolet end of the electromagnetic spectrum. These would be up in the very red end, the lowest energy.
So these emission wavelengths, these would be emitting at the lowest energy, longest wavelength. These would be emitting at the shortest wavelength, lowest to highest energy. Is everyone following me? So just make sure you remember that. And just this principle rule that you can't possibly break with respect to the wavelengths of light for fluorescence experiments.
So what we're going to see is the relationship for the electromagnetic spectrum. There's a little bit more detail in a minute. And then look at some fluorescent dyes that are very, very commonly used in biology. And in fact, you will have seen a lot of cells stained with these dyes even so far in pictures that you've seen on the screen. And then we'll talk about the application of antibody reagents and where does that come in with respect to fluorescences.
So let's take a look at fluorescence and the electromagnetic spectrum. So here it is, going from wavelengths. The ultraviolet wavelengths would be shorter than 400 nanometers. So ultraviolet, so beyond violet.
And the very red wavelengths would be in the range from 600 to 700. And here you see the relationship between wavelength, and then what the light emitted would look like. So if we're looking at here, what we would expect to see is if we've got a fluorophore and it shows fluorescence, we would be exciting the fluorophore in wavelengths in this region. And we would see emission in wavelengths in this region.
But a cardinal rule is that we excite with a shorter, higher energy wavelength and observe emission at a longer, lower energy wavelength. Now, there's very important dyes. Straight away the most common dye that you will see immediately in biology is a dye known as ethidium bromide. And here's its structure.
You can often recognize fluorophores. They have lots of rings fused together with lots of double bonds in them. This is the structure of a compound known as ethidium bromide. It's a dye that intercalates into DNA. And when the dye changes its environment from being in water to being snuggled in between stacks of base pairs in DNA, it changes its fluorescent properties and it becomes fluorescence.
So fluorescence isn't just the intrinsic shape of the molecule and what it looks like. It's very, very often related to what's around it. Why is that the case? It's because the excited state may behave differently in different environments. Maybe stabilize for a while, and that's why you might see fluorophores experience a change in their fluorescence as a function of their environment. Is that clear to everyone?
So the molecular environments, if I'm a fluorophore and I'm in water, I'm going to feel pretty differently in my excited state if I'm a fluorophore and I'm sitting packed between DNA bases. It's pretty dramatic when you see it.
So when you mix ethidium bromide with DNA, and it could be in a cell or it could be a lysate from a cell where you're capturing the DNA and trying to manipulate it in recombinant biology. That ethidium bromide will intercalate into the DNA. And it will light up as a bright orange dye.
So here, say we've got a gel that we've run DNA on. We might have a set of standards. And in other places, we're looking for the size of DNA. Remember that great experiment we saw where we saw how quickly small and large pieces of DNA ran through an agarose gel?
So here is what the DNA gel would look like if you soaked it ethidium bromide. So here's the gel as a ladder of bands. But then let's say you wanted to do some work on a piece of DNA and maybe ligated you see DNA pieces at different wavelengths that have different mobilities based on size.
We couldn't see the DNA directly. We couldn't pick it up. We couldn't visualize it. So we have to use ways to visualize it.
One way is to radio label it. Messy, we don't really want to do a lot of that if we can avoid it. The other way is simply to soak a dye into the gel. And the dye, because of the positive charge here, the counter ion gets displaced. And the positive charge gets attracted to the DNA and associates with it quite tightly.
So that would be a way that you would observe DNA bound to dye in a gel. And this fluoresces a pretty long wavelength. So this fluoresces this bright orange that's actually at about 605. So you can see, this is really in the visible range. 605 would be right around here. And it has this bright kind of orange fluorescence.
And the wavelength that you would use to irradiate the dye on the DNA would be a shorter wavelength than 605. You will often have a prescription, excite at this wavelength so you observe at this wavelength. And these are fixed physical parameters for fluorescent molecules.
Now, ethidium bromide is a dye that can get into cells. And we can look at DNA within cells. And here's a picture of how it would look.
So here's the ethidium bromide. And here's a pair of stacked bases, this one and this one. And there he is, right in the middle. See that ring coming towards you is that.
And then here's this thing that slides straight between the bases and might cause a little bit of a bulge. And we would call this a DNA intercalator. It slides into the DNA. And you can see over here, the structure of DNA.
So you could picture ethidium bromide sliding between the bases. Now, there's a big problem here. Because you can't use DNA ethidium bromide. It's pretty toxic. Why would it be toxic?
Well, no, it's not the bromide. It's the fact, more, think of what the dye does when it gets to the DNA. What would that do to things like replication and transcription? It just kind of messes it up.
And so these are toxic dyes that can only be used in fixed cells to do observations of cells. So we use it a lot. We need to be careful of it because if it gets absorbed through our skin, it could get into our cells. And it could interfere with replication and other cellular processes. Because it would accumulate on our cellular DNA.
And in fact, the interesting thing that a lot of molecules that actually have these sort of flat, pancake shapes with lots of double bonds are actually pretty important in biology. Because they end up being chemotherapeutic agents.
So what I've shown you here is what's known as an anthracycline structure. I believe this is adriamycin, I could be off. But it's a natural product that's isolated from bacteria. And it has this structure that also makes it a DNA intercalator. And it's used as a cancer chemotherapeutic agent because it interferes with cell division and proliferation.
So we actually exploit that property. But only with cells that we want to kill or stop dividing. So you could picture, well, I don't want to use something that's going to interfere with cells if I'm doing live cell imaging. Because I'm going to have trouble with the properties of the cells.
So fluorophores are great, but you've got to worry about them because they can get transferred through the skin, through cellular membranes because they're often quite greasy. And they can get in and interfere with essential processes of DNA. So because of this, there's been quite a revolution in the work done with DNA binding agents that bind a little differently and are way less toxic.
So I want to describe to you a series of dyes that are known as DAPI and HOECHST, H-O-E-C-H-S-T. This was, I believe, discovered in a bio company in Germany. And these are different kinds of dyes that fluoresce on binding to DNA. So they are still useful in that same context.
You can in fact substitute ethidium bromide with these dyes. But they bind to DNA pretty differently. And I want you to take a look at these pictures. So here in green and blue-- and apologies to the color blind-- you see a molecule of this compound here bound to DNA.
So it's pretty clear it's different from intercalation, right? You can see it's more sliding around one part of the DNA. And when those molecules bind to DNA in water, they don't fluorescence. When they bind to DNA they fluorescent an intense cyan blue. So that's at a shorter wavelength from the ethidium bromide.
So taking a look at this structure, does anyone want to explain to me how the molecules might bind to DNA? We wouldn't call it intercalation. We know intercalation is perpendicular to the axis of the DNA. So where, looking at this, do you think these bind?
A while ago when I was talking about the structure of DNA, I like to think of DNA as having two grooves, two places where things combine to it. And that's a minor groove. And then this big trench is what's called the major groove. And certain molecules bind in one groove. And other molecules bind in the other. Where do you think it's binding? Just by inspection.
Yeah.
AUDIENCE: Looks like it's in the minor groove.
PROFESSOR: That's correct, it's just snuggled just perfectly in the minor groove. If it was in the major groove, it would be swimming around in that groove. It's almost too big.
So what's really cool about these dyes is they slide in between into the minor groove. And they also make some contacts with the phosphodiester backbone. But it's not that they're dancing on the phosphodiester backbone. They're literally in the groove. But there's some opportunity for electrostatic interactions.
And so these compounds would be known to bind in the minor groove. And in fact, they bind in particular regions of DNA where there's AT, not GC. Those are the places where there's just the pair of hydrogen bonds instead of the trio. That's just their habit, their personality.
So chemistry was very important here. You had a good dye, but it was toxic. But improved dyes came along that could be used in living systems that are not toxic. Because if you bind in those grooves and you're dissociating easily, you're not going to interfere so much with replication. Does that make sense?
So it's a weaker force. It's not going to have a big detrimental effect. Now, I moved this slide up. I realized he was in the wrong place in the deck. This is just an application of the DNA minor groove binder CEOCHST.
And in this case, we're looking at three cells. These two are not actively dividing. But take a look at this cell, it's actually clearly in the state preparing for cell division. And what you can see here is that in the nucleus, the DNA is pretty diffuse before things really start to condense and line up for DNA replication and cell division.
And what's intriguing to me is that this rather diffuse blue dye, that's probably a bit more loosely associated with DNA, becomes much clearer and brighter when the chromosomes are in the state they're in for cell division. So you can see them here.
And one question here. So, if you're looking at cells, you're trying to observe cells, where else in the cell are you going to see DNA? So we can see the nuclear DNA, we can see what stage of the cell cycle it's in-- Yeah, Carmen.
AUDIENCE: The mitochondria.
PROFESSOR: Yeah, in the mitochondria. So you could also spot that within the cell if you're at a sufficient amplification. So you know that there's not DNA running around everywhere. It's literally in very specific places with the cell.
These dyes will bind also to other nucleic acids. But they don't bind so well. Because those don't have the really repetitive, double stranded nucleotide structures. But there are other dyes that bind much more specifically to RNA. But we won't discuss them.
So nucleic acids seem to be something that we can definitely pinpoint with fluorescence. We can see where it is. We could follow cell division. We could look to see the progress of cell division.
For example, upon adding things to a cell, can you see-- remember very, very early on, we showed you movies of cells dividing. You could do that with this kind of dye because it's a non-toxic dye. So, great, so far, so good.
So the key thing, though, about biology is we have so many other entities within a cell that we want to be able to track and monitor. And what we needed, what is absolutely essential are reagents to do that. So I want to talk to you about biological tools of monitoring.
And you know what these are? These are antibodies, monitoring proteins. And in fact, you can also coax antibodies to recognize carbohydrates. So I'm going to just put these here.
But they're a little bit harder to bind to antibodies. But nevertheless, those are useful. So we're going to talk now about antibodies, which are agents of the human adaptive immune system. And how they have been exploited intensively to study biology.
Now, what you will learn from Professor Martin in two or three lectures time is much more about the nuts and bolts of the immune cells, of the immune system. And how it mounts a response to disease and other features. I'm going to focus completely on the technological side of antibodies and how they are useful reagents to study biology.
Because if you want to recognize a protein in a cell, you need a particular entity that will bind to that protein and show you where it is through some kind of signal, for example, fluorescence. So what I want to do is give you the minimal description of antibodies so you can understand this. But later you're going to revisit it in a bit more complicated venue.
But for the time being, I'm just going to talk about B cells, which are cells that produce antibodies. And I'm going to talk to you about how they recognize their targets. Because what we have in the adaptive immune system is an amazing system where you can do combinatorial biology and basically recognize any target entity you're interested in.
So let's take a look at this, keeping in mind that this is us exploiting biology to make reagents to do biology. It's kind of a cool sort of cyclical process.
So the cells of the hematopoietic immune-- sorry the hematopoietic system, those are the ones that are important in blood cells, form a lot of different-- wait a minute, I'm on triple-- triple tools here. There are a bunch of different cells that are produced from the pluripotent hematopoietic cells. They're either the white cells or the red cell types.
But what we're going to focus right in on are the B cells. These are the cells of the immune system that produce soluble antibodies, OK. And when you challenge a B cell population with a foreign entity, the B cell population will go into gear to produce antibodies that very specifically recognize that foreign target, because in the human adaptive immune system, that might be a wonderful tool to get rid of that foreign entity.
So we're going to focus exclusively on the B cells and the way that they mature to produce soluble antibodies, those are down at the bottom here, based on what they've been challenged with. So what this little schematic shows you is you have a bunch of different B cells. And there's something you want to recognize. Let's just say it's a molecule-- an EGF molecule, a cytokine.
What you might do is challenge this population with the cytokine. Only one B cell type will bind to the cytokine. And then that will get amplified. And then you will end up with B cells that produce a lot of an antibody to a cytokine such as EGF. I'm truly dumbing this down. I just want you to get the gist of it for the purpose of this discussion.
Now B cells adopt a very classical shape. And I'm just going to show you the quaternary structure of a B cell-- of an antibody in linear form here. So it doesn't look very exciting right now. There are two light chains, which I've just shown in schematic form. These are just polypeptide chains. And there are two heavy chains.
All right, so that's their basic structure. It's held together in a stable quarternary structure with this complex. And there may be disulfides across and throughout the structure. Now what's so special about antibodies? They're pretty big molecules. The molecular weight is pretty high. But the key thing about antibodies is that the majority of the structure stays fairly constant.
So I'm just going to-- so this doesn't get modified when B cells mature. But another part of the structure is variable. And when B cells mature, there's loads of rearranging in that variable section in order that it adapt to bind to target. So what you've seen here is that the target-- see if this little fellow works anymore. No. Ah.
What you see here is the light chain in green, heavy chain in blue. And it's a double version of it. We always draw antibodies as this V shape. And an antigen-- you've heard this word before-- it's a foreign entity that's foreign to the immune system.
The antigen binding site is right here at the tips of the antibody. And I think the picture up there is kind of clearer. Let's put that forward again. And you can see very specifically the structure. The C's designate constant regions. See C all the way through here. And V's represent variable regions, which I've shown you. And at the tip of the V's are the antigen binding sites.
So you're going to see more about antibodies in the immune system. But what you want to accept is that these are biological macromolecules that particularly evolved to recognize target antigens. And you can use them reliably as biological reagents.
All right, so how do you achieve the diversity? There are hundreds of thousands of different antibodies in the human system. If we had a gene for every single different light chain and every heavy chain, you know, our DNA would be completely swamped by being dedicated to the genetic material for antibodies. So instead there is a particular system which provides little portions of the DNA structure that are in little pieces of variable components that can get zipped together through transcription and slicing events to give you a bunch of antibodies that have different variable regions. And this is what's known as the BDJ system. And you'll hear more about that from Professor Martin.
So basically what you want to think about is it's a combinatorial system to take little pieces of DNA into a super molecular structure to give you antibody combining sites that can recognize virtually any target. Anyone got any questions here? I'm seeing a few worried faces. Somebody ask me a question if you feel I could clarify a component of this or are you OK with this? Anybody?
OK, I'll move forward. So we talked about antibodies. When you get a population of B cells that produce antibodies to a particular target, these may be what are known as polyclonal-- a polyclonal-- sorry, I should have written that up here-- a polyclonal antibody, as might be suggested by the name, is an antibody-- let's say it recognizes a molecular entity. So the antibodies-- I'm going to draw them as little y shaped molecules-- may recognize different parts of the antigen. So antibody A, B, C recognize different parts of the antigen. Those would be polyclonal. It would be a mixed bag of antibody molecules that shows specificity for a target.
But people also tend to use a great deal of monoclonal antibodies, because they are a lot more specific. So if you had a selection of polyclonal antibodies, a monoclonal antibody would be a single population that recognizes a single antigen or epitope in your antigenic molecule. And the way those are made is through the engineering method where you fuse spleen cells with myeloma cells. And then you get a hybrid-- what are known as hybridomas that produce very specifically just monoclonal antibodies.
So that's a bit of background there about the antibodies. You'll see it on the rerun when Professor Martin talks. But I just wanted to give you a bit of exposure to this.
So let's now look at how antibodies can be useful. So let's say you want to visualize in a cell-- let's move straight to a real targeted application. We want to make an antibody that might recognize actin and a different antibody that might recognize tubulin to take a look at cells through the use of antibody structures.
The way you generate antibodies is through laboratory animals. And very commonly we use either mice or rabbits for antibody production. The rabbit is used when you need a lot more antibody material. The mouse will satisfy for some experiments.
So the way you make antibodies is by injecting. So this would be the foreign agent or antigen that you want to make an antibody to, which would normally bind at the variable region of this immunoglobulin molecule. You inject the mouse with that antigen. Excuse me-- that's a rabbit. You know, I haven't been in biology very long. But I can tell that's a rabbit, right?
Anyway, so you inject the rabbit with a human protein. If you-- what would happen if you injected the rabbit with rabbit actin or with rabbit tubulin? What would you-- would you expect to see a response? No, right? Yeah. Because the organisms are adapted not to recognize their own proteins unless there's some disorder like an autoimmune disease.
So you would inject the rabbit with a human epitope, so for example human actin, generate antibodies with a specificity for actin, and then you would label those antibodies with a fluorescent marker, so you could track it or follow it through chemistry. Alternatively, you might want to make a different antibody for tubulin. And then you would differentiate the antibody against tubulin from the antibody against actin by labeling it with a different color fluorophore.
So you've really got two types of macromolecule that can be interacted with a fixed cell and recognize those two macromolecules within the protein. So what's important here is that you don't use the protein from-- if you want to study human cells, you use antibodies produced in rabbit or mouse. If you want to study rabbit cells, you could produce it in different organisms. You're not going to produce the antibodies in the same host.
Nowadays-- yes.
AUDIENCE: How do you know that you have the correct antibody, like if that is the right one?
PROFESSOR: Right. OK, so you would prepare the-- you would do them. There's a lot of screening takes place. So you inject the mouse or rabbit. And then you collect the serum. And you look for whether the serum gets enriched and enriched in antibodies that recognize the target. So you'll see an increase in what's known as the titer.
And then once you get a high titer, you'll-- unfortunately, you can just collect the serum or depending if you want to make monoclonal, you'd have to sacrifice the animal to get the spleen cells. But then you collect the antibodies through an affinity method that's directed just at antibodies. Then you've got your population. And you can throw a fluorophore dye at it and chemically label it. So it's a good point there.
There's a lot of work being done now with antibodies from different organisms, in fact, you'll see them from camels. And they're also ones from shark. And the reason why they're kind of interesting is that they sort of have mini antibodies that are much more useful for technology.
So let's see what we can do here. We can do fluorescence experiments. In order to do this with an antibody. And this is going to highlight a shortcoming of antibodies. You may take a cell that you want to observe different proteins in that cell, you have to fix the cell to make it permeable. Why do I need to make the cell permeable in order to use these antibody reagents?
Do you think the antibodies are just going to cross-- DAPI gets into a cell easily. But what about antibodies? Can they cross the plasma membrane to get into the cell to label a target? What do you think? Who says yes? Who says no? Good. Thank goodness. OK. You guys don't say much. But I know you know the answer here.
They just can't float into cells. They're too large to get into cells. So you have to fix the cells on a glass side and permeabilize them, for example, with methanol so that the antibodies can gain access to all parts of the cell. So here is a bright field view of cells. That's a little bit more specific. That's OK too. But this is what I really want to show you. This is what you could achieve with DAPI and an antibody to the actin and an antibody to tubulin.
And you can look at the various colors of the fluorescence emission. And you see here what you've got is an anti-actin antibody with a red dye. And you can see that at the perimeters of the cells. You've got an anti-tubulin antibody with a green dye. And you can see the filamentous structure there. And then you've got DAPI staining bright blue where the nuclei are.
So you have three unique labels that fluoresce at different wavelengths and you can directly pinpoint things. So fluorescence is extremely valuable for looking a biological systems, because we don't have a lot that fluoresces in the body. So a cell in general, if you irradiate it with light, you won't see any major fluorescence at all.
So these reagents that you use to study biology, if they fluoresce, you've got unique signals where you can look at really complicated cells that may have thousands and thousands of proteins, sugars, nucleic acids, and very specifically see things by fluorescence because the fluorescence is a unique signal in biology relative to the intrinsic fluorescence of proteins or rather macromolecules.
We have tryptophan and tyrosine, a couple of amino acids. They fluoresce, but it's so dim. It's nothing like this. You wouldn't see those kinds of signals at all. It's really what we call extrinsic fluorophores, fluorophores from outside that shine very, very brightly. Many of these fluorophores shine so brightly they can be used to look at in single molecules-- professor Martin described to you single molecule DNA sequencing. That actually exploits very bright fluorophores that is so bright that you can see just a few of them in one place very, very clearly.
OK, how am I doing? I just want to actually leave you with something that's another technology. So you could almost picture-- with all of what we've seen so far, you could almost target any cell with an antibody that's specifically raised to a particular protein that's within the cell. So we can see-- in the next class we'll discuss what the limitations of that are. But we've already talked about the fact that we have to use antibodies with fixed, not living anymore, cells. So they are really dyes that can only be used in that way.
The other place you can use fluorophores is for labeling DNA and from looking at DNA. And a particularly important technology is known as a DNA microarray has anybody heard of these? Yeah. So DNA microarrays absolutely exploits the complementarity of DNA sequences. So if you want to probe for a particular sequence of DNA, you would make the complementary strand and label it with a fluorophore. And new could, out of thousands of DNA stretches, literally light up the stretch of DNA that's complementary to your target.
And so DNA microarrays-- what you see here are just the size of just a microscope slide. On this slide through arranged technologies, you can literally spot 40,000 distinct sequences of DNA in grids to recognize. So these DNA microarrays can be used for profiling genetic material or for profiling not just DNA, but RNA, and we'll see how at the beginning of the next class, in order to probe for particular stretches of DNA that might be disease related and have single nucleotide polymorphisms.
So in order to actually just give you a little bit of a warm up to that, I want you to go-- I'm going to put a link to this in the web site. And it actually just shows you a virtual running of a DNA microarray experiments and how you can use it to profile disease states and cells versus healthy cells. And then at the beginning of the next class, I'll describe how you get information out of DNA microarrays.
But at the end of the day, you're always using the fluorophores as the probes for where certain things are, OK. And the thing to remember here. Fluorescence is a magnificent tool. We can use fluorophores on their own. We can use fluorophores as attached to antibodies. And the DNA microarray experiments show you how you can use fluorophores attached to DNA sequences, OK. And I'll see you in the next class.