Flash and JavaScript are required for this feature.
Download the video from Internet Archive.
Description
Using the example of aniridia, which disrupts formation of the iris, Professor Martin describes how to clone a gene that's responsible for a disease. He covers the techniques of positional gene cloning, genomic mapping, cDNA libraries, and hybridization.
Instructor: Adam Martin
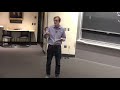
Lecture 18: SNPs and Human ...
ADAM MARTIN: And so I just want to say a couple sentences about DNA sequencing, just to finish that up. And so you'll remember this slide from last lecture. And remember, the way this Sanger technique works is to set up four different reactions where each reaction has a different one of these dideoxynucleotides. OK, so there's four reactions, each with different dideoxy NTP.
And I brought along a gel that I ran a while ago, which is basically-- it's from sequencing gel, and you can-- I'll pass this around so you can take a look at it. So the four different lanes for each sample are the different dideoxynucleotide reactions. And what I want you to notice as that's passing around and you're looking at it is that the different reactions with the different dideoxynucleotides give different patterns of DNA fragment lengths. So there are different patterns of fragment lengths. And the different patterns are based on the fact-- this is based on the sequence, the sequence of the template, OK?
And so if we look at the example up here, what you'll see is that in this banding pattern for dideoxy TTP, you see that there's a really short fragment at the bottom there, and so that fragment indicates that there must be an A in the template sequence. The next fragment up would be this one in the dideoxy GTP lane, and that indicates that one nucleotide beyond this A is a C position, and so on and so forth, such that you can sort of order the fragments and see which reaction has a fragment and then read off a DNA sequence. OK, so conceptually, that's how you would read off the sequence of a given strand of DNA, OK?
So you might be wondering, if now, we just read off sequence as a series of colors, why am I even introducing this technique? And the reason is because I think it's important for you as potentially future scientists to know that when you're faced with a problem, how you might discover something new. And I see the Sanger method of DNA sequencing as a really clever and elegant way in which Fred Sanger solved the problem of DNA sequencing, and while we don't necessarily do it that way today, it still illustrates a concept that's important, the concept of chain termination, and I think there is something to be learned from this older technique, even if it's not exactly how we sequence DNA today.
So for today's lecture, we're going to continue on our quest to basically clone a gene that's responsible for a disease. And so we started this in the last lecture. And I guess one thing we would want to start with is a disease, so I'm going to introduce to you now a disease called aniridia. And in order to clone the gene for a disease, it has to be a heritable disease, in this case, because we're going to use linkage analysis to identify it.
So aniridia is a disease that's an eye disease in humans. It's a rare eye disease. So I want to show you a bit of an example of this eye disease. The way this disease manifests itself is it's basically the affected individual has an eye that is lacking an iris.
So I'm going to show you what this looks like. If you're squeamish or don't like weird eyes and you don't want to look, you can look away. But I will show you affected phenotype in 3, 2, 1, OK, everyone looking who wants to see weird eyes. OK, good.
So that is a individual that has aniridia, and also this one. So you see there's no clear iris in these eyes. And this disease is associated with other abnormalities of the eye that severely impair vision. And this is an inherited disease, and this is a pedigree from a family or series of families where the disease is propagating. And so anyone have a suggestion as to what mode of inheritance this is?
Anyone want to rule a mode out? Rachel, you have an idea?
AUDIENCE: I was going to say X-linked dominant, but [INAUDIBLE]
ADAM MARTIN: OK, so let's take X-linked dominant. So if it was X-linked dominant, then this male would have an X chromosome with the dominant allele of the disease and should only pass it to his females. So I don't think that it would necessarily be X-linked dominant. Anyone else have an idea? Yeah, Georgia?
AUDIENCE: Autosomal.
ADAM MARTIN: Autosomal dominant. I like autosomal dominant. So in this case, you see you have an individual with the disease and they marry into a family with no history of the disease.
One thing I'll point out, for many of these diseases, they're extremely rare, so if you see sort of a family tree where there's no instance of the disease, if it's a rare disease, it's likely that these individuals are not carriers. And so in this case, if you assume that this person doesn't have any form of the-- isn't a carrier for the disease, then this cross here resulting in about half of the individuals affected with the disease, that would be a characteristic of an autosomal dominant disease. So everyone understand my logic? Yes, Carlos?
AUDIENCE: What are-- why is two and that looks like three on the slide, why are they crossed out?
ADAM MARTIN: I think they're deceased. Yes. OK, so let's say you have a pedigree. You have pedigrees, you're able to try to link this marker to-- or the disease phenotype with various molecular markers, which we discussed in last week's lectures, then you're on the way to performing a process which is known as positional gene cloning.
And what positional gene cloning is is it's basically cloning a gene and a allele that's responsible for a disease based on its position in the genome, it's position in a particular chromosomal region. So it's basically cloning a gene based on its chromosomal position or its chromosome position. And the first step of positional gene cloning would be to establish maybe what chromosome it's on. And a straightforward way to do this, as we've basically been discussing almost from when I started lecturing, is to create some sort of linkage map or do linkage mapping to identify, in the case of humans, molecular markers that this disease allele is linked to.
And remember, in last week's lecture, we talked about a number of different polymorphisms that are present in the human genome that we can use to establish linkage with a given phenotype. In this case, it's a human disease. And we talked about this example for a microsatellite marker. And in this case, we talked through this example of how this dominant allele, P, is linked to this microsatellite allele m double prime, because if you look at the pedigree here, all of the affected individuals here contain this m double prime sized fragment for this microsatellite.
Another thing to notice here is you can see that this couple has been faithful to each other, because basically, each of the children have an allele from the father and an allele from the mother. So you can see that type of-- you can see that using this type of molecular marker as well. OK, so you establish linkage. So linkage mapping establishes the chromosome position of a given allele and the gene.
And this chromosome position sort of gets maybe in the right country, but you still have a long way before you get to the specific street address. And so you have to then sort of narrow it in to identify a smaller region of the chromosome that could possibly contain this gene. And so what you would do is go from this linkage map, where you maybe identify the position of this gene within a couple map units, to this next resolution of map called a physical map, OK?
So we go from the linkage position to the physical map of the chromosome. And the physical map, as the name implies, is when you have physical pieces of DNA that are present in this region of the chromosome. So the physical map means you have cloned, so recombinant pieces of DNA, cloned pieces of DNA which encompass a given chromosome region. So these are encompassing a chromosome region.
OK, so how would you get a piece of DNA that sort of is in this region? How would you start? How would you start fishing for that DNA?
So you've gone through the process of linkage, you've identified sort of a polymorphism that is linked to the disease allele. How would you go from there to getting a physical piece of DNA that is present in that region of the chromosome? So let's think back to-- Jeremy, did you have an idea?
AUDIENCE: Start by using PCR to just amplify that chunk. [INAUDIBLE]
ADAM MARTIN: And what primers, I guess, would you use for the PCR?
AUDIENCE: Depending on which chunk you're trying to get, you'd use [INAUDIBLE]
ADAM MARTIN: OK, so Jeremy is saying if you knew the sequence, and I guess if you're doing this microsatellite analysis, you had primers that recognize a sequence at a given genomic position, so you actually know something about the sequence because of this polymorphism, so you can use that knowledge to then look for this sequence. And you could even look for the microsatellite in a DNA library. OK, so you have cloned pieces of DNA, and you're going to start with-- I'm going to swap this.
Your starting position could be one of these polymorphisms in the sequence around it, which you already know. So let's say you had this microsatellite marker. You could then-- what I'm drawing here is a piece of genomic DNA. So this is genomic DNA.
I'm just drawing the insert. This would be recombinant DNA. It would be present in some vector or plasmid. But if you can identify the sequence that contains this microsatellite marker, then you would have the microsatellite, but also the surrounding DNA, OK?
So that sort of anchors you at a given position. Now, you don't know if your gene is in that piece of DNA, but you know that it's linked, and so it should be around that piece of DNA somewhere. And so it's unlikely your gene is going to be on this small piece of DNA that's cloned. This is probably just a few kb, and you could still be very far away from this, but that serves as a starting point from which you can go from to get more and more pieces of DNA such that eventually, you have a bunch of pieces of DNA that are going to span the entire region.
So the way you identify other pieces of DNA is you could start with a piece of DNA maybe at the end of this insert and look for other inserts that are not identical to this piece that also contain this piece here. So that might get you a piece that's overlapping, but extends farther than your initial piece. So now you've moved slightly farther away from your starting point, which is this starting polymorphism.
Then you could choose maybe another DNA sequence here and look for a piece of DNA that, again, is extending a bit farther out. And so you can see how iteratively, you can get farther and farther away from this starting point that you know your gene is linked to. And this process of going sort piece by piece and clone by clone away from a starting position is known as a chromosome walk.
And you can do this bidirectionally. So you could also start with a sequence of DNA here and look for a clone that goes the other way. And you can see on my slide up there, you can see that in this case, they've taken a one map unit region of the chromosome and they're illustrating physical pieces of DNA that are overlapping that encompass this entire region. So this could be much bigger than the amount of DNA that would fit in one of these clones in the bacteria, but by sort of identify overlapping clones, you get the entire region. And what this is called here, because these pieces of DNA are contiguous with each other, this is known as a contig. Yeah, Jeremy?
AUDIENCE: So would how you get the-- once you find one of those pieces, how do you get the primer for the end of it to start? Do you actually sequence each of these pieces of DNA?
ADAM MARTIN: You could sequence it, or you could use a technique that I'm going to talk about at the end of my lecture, which I'll come back to. So nowadays, you'd probably just sequence it and then maybe look for that in another clone. But even before we could sequence DNA in entire genomes, you could do that type of experiment by using a technique called hybridization, which I'll come back to.
OK, so the question in this chromosome walk then becomes, how do you know when to stop? Because you could do this for a very long time, but it might not be useful. So you have to know when to stop, and you need to know when you arrive at the gene that you're interested in, which would be the gene that is responsible for the disease.
So another way to phrase this question is, how do you know when you have an interesting gene on one of these fragments? So let's say this is an interesting gene here. How do you identify interesting genes? So now, let's talk about identifying interesting genes. Anyone have an idea for how they would-- what criteria they would use to define a gene as being interesting here?
I mean, one could say that all genes are interesting. If it's a gene, it's interesting, right? How might we define whether or not there's a gene even there?
It could be-- there could be a gene-- how would you define a gene? Can someone define for me a gene? Yeah, Miles? Is it Miles? No? Malik, OK.
AUDIENCE: [INAUDIBLE] that would create a starting and stopping point. So like [INAUDIBLE]
ADAM MARTIN: So you'd look for a piece of DNA that has a start and a stop codon? So you'd look for an open reading frame, basically. Yeah. You could look for an open reading frame.
And so I totally agree with Malik there. And another criteria you could use is if it's encoding a protein, at some point, it also must have been transcribed as an mRNA. And there are some genes that are transcribed as RNA but don't make a protein, and they're often involved in coding or in regulation of gene expression.
So I'm going to-- I'm going to say, is it transcribed? So is there some transcript that's made? And specifically, is it transcribed in the tissue that we're interested in?
So if we're talking aniridia, we might be looking for genes that are being expressed or transcribed specifically in eyes. You're looking for something that might be expressed in the eye. If it's not expressed in the eye, that gene's going to be much less interesting to you because the phenotype of aniridia is clearly in the eye.
OK, what might be some other criteria here? Well, one criteria might be, is there a conserved gene that has an interesting function that's maybe similar to the disease related phenotype? So is there a conserved gene with an interesting function?
And to take this example of aniridia, let's say you're doing this chromosome walk, and you identify a gene, maybe you sequence part of this clone, you get a string of sequence, and you realize that the sequence that you get is related to a gene from a model organism, and maybe that gene is called eyeless. If you've identified a region of sequence in a human, in the human genome that's mapping to an eye disease gene, and you find out that in that region, there is a conserved gene called eyeless, might be a very interesting gene for you. So eyeless is a gene.
So here's a normal fly. You see it has that bright red eye. The eyeless gene, when mutated, results in a fly that now just doesn't have a white eye, but has no eye altogether.
So it turns out that the aniridia gene is the homolog of the eyeless gene in flies. That's not how it was identified initially, but nowadays, there's a lot of information in model organisms. And so if you're sort of trying to identify a gene, and you see that there's a gene in the neighborhood you're looking at with a function that's related to a gene like eyeless, which has a clear sort of analogy in terms of phenotypes, then that's going to increase your interest in that gene.
So I'm going to come back to this point here, which is how do we determine whether a piece of DNA that's on one of these inserts that we're getting as we walk across the chromosome, how do we know whether it is transcribed or not? And to get at this, I'm going to introduce you to a concept which is important in and of itself, which is the idea of cDNA. So cDNA.
And specifically, I'm going to show you how one would make a cDNA library, which is basically a library of different cDNAs. And so what cDNA is, as shown up there on my slide, a cDNA is complementary DNA. It's complementary DNA, meaning that is the complement of an mRNA transcript. This DNA is the complement of an RNA or mRNA transcript.
One thing to watch out for is it's not complimentary DNA. So this is MIT. This is a no compliment zone, so I don't want to see any complimentary DNA. All right, so let's think about complementary DNA.
So remember, we've talked about the central dogma and how DNA encodes for RNA, which encodes for protein. And so the information flows from DNA through RNA to protein. But there are some specialized cases in biology where this information flow is reversed. So there can be a reverse of information flow where information flows from RNA to DNA.
OK, so that's pretty cool. Where does that happen? Well, there are viruses, such as retroviruses, one example of a retrovirus is HIV, and the virus life-- the virus genome is a single-stranded RNA molecule, and the life cycle of the virus is that inserts into the host-- the host genome, which is double-stranded DNA.
For a retrovirus to do that, it needs to take its RNA genome and make double-stranded DNA in order for it to insert. So this is an example in biology, which is basically breaking the rules that we talked to you about earlier in the semester. Also, there are retrotransposons which do a similar process, going from an RNA molecule to double-stranded DNA. So this is a specialized case, and it's interesting, and we can take advantage of it to basically clone and identify mRNA transcripts.
OK, so I'm going to tell you how to make complementary DNA, and I'll go through a series of steps. The first step is we want to make complementary DNA of mRNA, so we need a way to purify the mRNA. So anyone have any idea how to purify mRNA?
First, we could maybe draw an RNA molecule here. What are some salient features of mature mRNA? Yeah, Carlos?
AUDIENCE: It'll have the five-prime cap [INAUDIBLE] phosphate.
ADAM MARTIN: Yeah, it'll have a five-prime cap. Anything else? Jeremy?
AUDIENCE: Poly-A tail.
ADAM MARTIN: It'll have a five-prime cap and a poly-A tail. I'm going to take advantage mostly of the poly-A tail here. So here, we have a poly-A tail. OK, how might we use that poly-A tail to purify mRNA? Natalie?
AUDIENCE: Well, you can add a [INAUDIBLE] because you know they're [INAUDIBLE]
ADAM MARTIN: Mm-hmm. What sequence would you use?
AUDIENCE: [INAUDIBLE]
ADAM MARTIN: Yes. So Natalie has suggested using poly T, which she said would stick to this poly A tail because of base pair hybridization, OK? So let's say we have a bead or some type of resin with dTs hanging off of it. So I'll draw a few of them, but you'd have maybe a lot of them sticking off, OK?
So you have a bead with pieces of DNA, all of which are poly dT hanging off of it. And then these poly dTs, if you add cytoplasm from cells, the mRNA in that cytoplasm is going to stick to this poly dT bead, and it will stick with a higher affinity than other things that are non specifically sticking to the beads, and you can wash these beads with buffer and salt to get rid of everything that's non-specifically sticking to the bead, and then you're left with just a bead that's enriched with mRNA, which is what was specifically sticking to this, OK?
So you could purify-- you're purifying the mRNA based on its affinity for a poly dT, OK? So then you're going to have enrichment of mRNA in your sample. And so then once you have your RNA, you're going to want to somehow go from RNA to DNA, OK? So the next step will involve somehow going from RNA to DNA.
So let's draw our piece of RNA here. Here's our RNA. It has a poly A tail so it's mRNA. There is 5 prime. OK, so now we need to take advantage of a trick. We can still take advantage of dT because we can use this as a primer because polymerase usually requires some primer and a three prime hydroxyl in order to extend.
Now, can we use DNA polymerase to extend this primer? Jeremy is shaking his head no. Why?
AUDIENCE: Because DNA [INAUDIBLE]
ADAM MARTIN: Exactly. So what Jeremy is saying is DNA polymerase is a DNA dependent DNA polymerase, OK? DNA polymerase can only use this if this is DNA here, OK? So we need a different type of enzyme, essentially, in order to make DNA from RNA, and luckily, molecular biologists-- actually one of whom was here at MIT-- discovered this type of enzyme, and it's called reverse transcriptase.
Reverse transcriptase. This is an enzyme that's encoded by retroviruses in order to make double stranded DNA from RNA, and that allows the retrovirus to insert into the host genome, OK? And what reverse transcriptase is is it's an RNA dependent DNA polymerase, OK? So it takes RNA as its substrate, and then it synthesizes DNA on the opposite strand, OK?
So this is an RNA dependent DNA polymerase. OK, so if you add reverse transcriptase to mRNAs that have these dT primers, then what you get is a new strand, which is DNA here. This is the strand of DNA. And then you have a strand of RNA opposite it, OK?
So at this step, you have a DNA RNA hybrid. So this is a DNA RNA hybrid. Let's see. Reveal some more of this. This is the process which I'm basically outlining on the board. So then you want double stranded DNA, so you don't want this strand of RNA that's down here, so you have to get rid of it. So you would degrade the RNA, and this is done using another enzymatic activity, which is derived from reverse transcriptase, which is termed RNAs H activity.
So you can add an enzyme RNAs H, which RNAs H takes this DNA RNA hybrids and degrades the RNA part of it, OK? So this is going to degrade the RNA strand. And if you degrade the RNA strand, then you're left with a single strand of DNA. So you have single strand of DNA here, and now what you need to do is to synthesize the second strand of DNA. So you need a second strand synthesis.
And so you need, again, a primer in order to prime the synthesis here. So there are a variety of ways to do this. You can add some type of hairpin, which is five prime here and three prime here, and then you can use either DNA, polymerase, or reverse transcriptase, which also can be a DNA dependent DNA polymerase to transcribe this strand here, OK? So again, you add polymerase, and now you've gone and you've generated double stranded DNA, OK?
So everyone see how we've gone from an mRNA transcript, and we've done the reverse of everything we just told you in the first half of the course because we've gone from RNA and we've made DNA, OK? But this will be really useful because now we have a stable piece of DNA that we can clone into a plasmid and we have a record of this transcript being present in our sample, and we can propagate that on and on, so we've cloned it, OK? All right, what's going to be special about this piece of DNA versus a piece of genomic DNA? Natalie?
AUDIENCE: [INAUDIBLE]
ADAM MARTIN: Yes, so Natalie suggesting that it doesn't have introns, and that's totally right. So this is not like genomic DNA, and what Natalie said is because mRNA is processed, the introns are spliced out, such the mature mRNA only has the axons, and so this piece of complementary cDNA is going to have no introns. How else is it different? Yeah, Jeremy?
AUDIENCE: It's not going to have promoters.
ADAM MARTIN: It's not going to have a promoter. Yes, Carmen?
AUDIENCE: It doesn't have [INAUDIBLE]
ADAM MARTIN: You might see a poly A and T sequence in the cDNA. Yes, that's true. OK, so you might have poly A, poly T. I'm going to focus on the other part from-- there's going to be no promoter, enhancer, regulatory sequences. Basically, it's got no sequence that's not transcribed, right?
The DNA is only going to have the part of the gene that was physically transcribed by the RNA polymerase originally. OK, so no non-transcribed regions. No non-transcribed regions, and Carmen's absolutely right. You will also have possibly a poly A or poly T sequence. OK, so when you get these cDNAs, you might have-- you have more than one mRNA in a sample like a cytoplasmic extract, so you're going to prime-- you're going to make multiple cDNA and different cDNAs will reflect different transcripts that are present in your sample, OK?
So you could have one clone that's one gene, another clone that's a different gene, and another clone that's another gene, and you could have thousands of clones of these different DNAs. What's going to be special about what types of genes are you going to get for I guess different tissues. Are they going to be the same or not? Yeah, Carlos?
AUDIENCE: [INAUDIBLE]
ADAM MARTIN: Exactly. You're not going to see-- if you've prepared a tissue and there is no gene being-- if one gene was not expressed or transcribed in that tissue, you will not get a cDNA for that particular gene in your library, OK? So the representation of genes-- the representation of genes in a cDNA library is totally dependent on what genes are being expressed, OK?
So this representation is going to be proportional to the expression level, and the more genes-- the more a gene is expressed in a given tissue, the more copies of cDNA for that gene you would see in the library, OK? So there's really a proportionality between the number of clones in a library and the expression level of a gene, where in the most extreme case, if this gene is not expressed at all, you're not going to see it represented at all in the cDNA library, OK?
And then a corollary to this statement is that if you make cDNA libraries from different cell types or different tissue types, the cDNA libraries are going to be different between those different types of sources of mRNA, OK? So in other words, different tissues give you different cDNA. OK, so there is the process.
So I went through most of the side. Yes, miles?
AUDIENCE: Is this a way you can determine what gene sequences are expressed in all cells? Because in certain mRNA strands across all tissue samples, those are basic cell functions and expressed in a [INAUDIBLE] organism?
ADAM MARTIN: So you're asking, if you grind up like an entire organism and if you get a cDNA from that library, could you tell if it's expressed in all different cell types? Even if you have one cell type that expresses a gene, if you grind up the entire organism, then you're going to have some mRNA that represents that gene. So I don't think it would be as an effective measure to determine the ubiquity of expression of a given gene, but in just a minute, I'm going to give you a tool that would allow you to answer the exact question that you're asking, OK? Any other questions about the cDNA library? OK.
So I just wanted to mention that a comeback to this example I gave on the identification of the human CDK gene. So remember, we started with yeast that were mutant. They had temperature-sensitive mutants, and we transformed these mutants with a library, but I didn't really tell you what the library was. It was in fact the cDNA library from humans that was transformed into yeast, OK? And that's because yeast genes-- for the most part, they don't have a lot of interests, and so the yeast-- the machinery is not able to splice out the human interactions and human genes, OK?
And so this was done with a human cDNA library, which then encoded-- one of which encoded the cumin CDK gene, and that allowed Paul Nurse to discover the piece of DNA that encoded for the human CDK, OK? So I just wanted to kind of retroactively go back and sort of tell you how that experiment was done. OK, so now I'm going to get to my final point for this lecture, which is this final technique, which will allow us to determine whether or not a transcript is expressed in a single cell type or ubiquitously through an organism, and this involves a technique, which is known as hybridization.
And what hybridization is is if you're starting with a piece of DNA, you don't need to know its sequence in order to determine whether there are sequences that are similar or identical to it, because hybridisation is basically if you have some sequence and it's single stranded such that you have a DNA backbone but you have base pairs that are able to pair with their complementary bases and you can use a piece of single stranded DNA like this and you can label it such that if the labeled piece sticks to another piece that has identical or similar sequence, you'll be able to visualize it in some way, OK? So this is called-- you're looking for things that anneal or hybridize to a particular specific sequence.
So you don't need to know the sequence a priori, OK? You just need to have this physical piece of DNA, and you can use this single stranded piece of DNA to then fish for similar sequences, OK? So we could take a piece of DNA here maybe that's in a gene, and we could fish through a DNA library to try to identify a cDNA clone that has sequence identity to that piece of DNA, OK?
And the way this is done is to take a cDNA library. So each of these colonies here would express or have a different clone of DNA. You can then take a nitrocellulose filter, put it on this plate, which would stick the bacteria in place to that filter, and you could then lice the bacteria and denature the DNA, and then the DNA is stuck to the figure, but now it's single stranded.
You can then add your probe, which is labeled, and look for the colonies that this probe sticks to, and that would then identify a particular cDNA, which would identify whether or not a piece of DNA is expressed in a given tissue type, OK? So everyone see how that would work? So in addition to doing this on a nitrous cellulose filter, you can also do this in a tissue, and that's known as in situ hybridization.
And in this case, in situ hybridization, you're searching for mRNA in a section of fixed tissue. OK, and I have an example from this paper here, which is the paper this are cloned. In this paper was the cloning of the aniridia gene, and they identified a gene of interest, which is called Pax6 now, and they basically used a piece of DNA that they thought was interesting, and they did in situ hybridization in an organism, in this case, you see an eye.
This is an eye here, and the label Pax6 is labeled in yellow, and you can see how this transcript is present throughout the entire eye, right? And the way you would see if it's tissue specific is you look in other tissues and you wouldn't see this yellow label. So that's how you would determine if it's expressed in a specific tissue or ubiquitously throughout an organism.
OK, so this Pax6 gene. Oop. So I was going to ask, what do you think would happen if you hyperactivate Pax6 in humans, and this is one idea, but actually, I just made that up, or Stan Lee made that up, but actually, Stan Lee never in fact mentioned whether or not cyclops is a Pax6 mutant, but we can do a different type of experiment, which might be more ethical, which is we know there's a fly gene that's homologous to Pax6. And what we can do in flies is we can topically express this islets gene in non-eye tissues and see what happens.
OK so, this is pretty wild. This is my Halloween image of the class. So this is a fly where eyeless has been expressed all over its body. OK, so here you see there's an eye-- It's normal eye-- here. You can see there's now another eye growing in the front of its head. You can see here's an eye growing on this fly's back, and you can see the legs. There's eye tissue all over the legs of this fly, OK?
So this Pax6 gene, which is conserved from flies to humans is the master regulator of eye development, OK? And at least in flies, if you topically express this in other parts of the body, you get an eye. I should say these are not functionalized. They don't hook up to the brain the same way the normal eye does. So it's not like this fly can see out of the back of its head.
OK, that's it. I'm done, and good luck on your exam on Wednesday. We will see you here.