Flash and JavaScript are required for this feature.
Download the video from Internet Archive.
Description
The theme for the next few lectures is the cell division cycle. Professor Martin kicks off this series with a discussion on what needs to happen in a cell during the cell division cycle, and what checkpoints ensure the proper order of events.
Instructor: Adam Martin
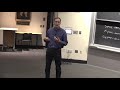
Lecture 23: Cell Cycle and ...
ADAM MARTIN: And so today and for the remainder of the week, the theme is going to be the cell division cycle. And so we're going to really talk about the cell division cycle in every lecture this week with the penultimate lecture talking about how dysregulation of the cell division cycle results in a pathological condition known as cancer.
OK, so here is now a cell going through the cell division cycle. It's entered into mitosis right now. And these guys here are the chromosomes of the cell. And you're going to see them line up at the metaphase plate. And eventually they'll be segregated to the two poles of the cell. And then the cell will divide along its equator.
OK, so I thought we could start today by just thinking about what has to happen in a cell during the cell division cycle. What has to happen during this process in order for the cell to replicate? Yes, Miles?
AUDIENCE: For all the [INAUDIBLE] all those have to be duplicated so that each cell has a starting number.
ADAM MARTIN: Mmm hmm. So Miles suggested the organelles have to be duplicated such that the daughter cells can inherit those organelles. And that's correct. What else has to happen? Anything else have to be duplicated? Stephen--
AUDIENCE: DNA has to be duplicated.
ADAM MARTIN: The DNA, the nuclear DNA, the chromosomes, have to be duplicated. So the chromosomes have to be duplicated-- duplicated. What else has to happen every cell cycle? What would happen to the size of the cell just when it divides? Yeah, Udo?
AUDIENCE: It would grow.
ADAM MARTIN: So Udo is suggesting that the cell has to grow, right? Because if the cell didn't grow, then cell division would make smaller and smaller and smaller cells. And so another thing the cell has to do during the cell cycle at some point, it has to grow in size. OK, and what's the final point of the cell cycle? What happens? What's kind of the goal of the cell cycle? Yes, Stephen?
AUDIENCE: Undergo mitosis.
ADAM MARTIN: To undergo mitosis. And so the cell has to physically divide, right? The chromosomes have to be segregated, and the cell has to physically divide. OK, so you can think of the cell cycle as the goal of getting all these events to happen is to get one cell to become two cells. So you need chromosome segregation. And you want an equal segregation of genetic material into two daughter cells after the cell divides.
OK, so today, we're going to unpack the mechanisms that allow a cell to do many of these things and how it's regulated. And one thing to think about is, what is going to determine whether or not a cell enters the cell division cycle and undergoes a division? What do you think are some things that cells would care about if it's trying to decide whether or not to divide?
So one thing a cell is going to care about in a multicellular setting is whether it's getting appropriate communications from other cells that are telling it to divide. And remember, Professor Imperiali told you about signaling. And one example was receptor tyrosine kinase signaling. And this is just a diagram showing you the RAS map kinase pathway.
And one of the effects of this signaling pathway is to promote cells to enter the cell division cycle in order to divide, OK? So this is in a multicellular organism, the signaling is important. For unicellular organisms, cells might care about whether or not there are nutrients present or whether or not the cell is of the right size, OK? So cells have to make the decision. I'm going to focus mainly on cell communication and how that might change the cell physiology.
And I want to start by just giving you a little bit of an overview of the cell division cycle. So there are four distinct phases of the cell division cycle. And I split them into two classes. There are phases where things physically happen to the cell. And those are S phase and M phase.
And so during S phase, S phase stands for DNA synthesis. And it's during this phase when the nuclear DNA is replicated, OK? And so for each of the action phases, if you will, there's some sort of machinery that's involved in changing the cell.
In the case of S phase, that would be DNA polymerase and helicases that mediate the replication. So helicases, DNA polymerase. And you remember from earlier in the semester when we talked about DNA replication all of the proteins that are involved in replicating a chromosome.
OK, the other phase where something really physical happens is M phase, which is mitosis. And during M phase, this is when the sister chromatids of each chromosome are separated to the daughter cells. OK, so this is when chromosome segregation happens. And again, during this phase, there has to be some sort of machine that gets activated at this phase of the cell cycle in order to, in this case, physically separate the chromosomes from each other.
And that machine in M phase is the mitotic spindle, which you'll recall is a machine that consists of microtubules. So these are microtubules. And in each cell cycle, these events have to happen.
But they have to happen in order, right? You need DNA synthesis before you segregate the chromosomes, right? So there has to be an order to this.
So these other phases are called gap phases. And there are two of them, G1 and G2. And events happen during these gap phases to help to ensure that things happen in the right order.
And so in G1, the cell has to decide whether or not to enter into the cell cycle. So some things to consider here, the one that I mentioned before is whether or not there are growth signals present. OK, so for a metazoan cell, it's important that the cell doesn't just divide without any regard to what's going on in the surroundings. There needs to be a communication between cells such that there's the proper balance of cell division in a tissue for specific cell types.
OK, so this G1 phase, this is when the cell-- if the cell goes from G1 to S, this is when the cell commits to the cell cycle. So G1 to S, this is the time when the cell commits to going through the entire cell cycle. So if the cell passes this G1 to S transition, then the cell has committed to going through the entire cell cycle.
OK, the other phase, this G2 phase, ensures that this type of quality control that happens, it has to ensure that the DNA is replicated before the cell moves on to mitosis. So you can think of G2 as a phase where there's a quality control mechanism and the cell cares about whether or not its DNA is replicated or not.
OK, so now I want to tell you basically the answer as to how this system works in a eukaryotic cell. And this system requires a level of control. And it requires a control system.
And what this control system does is to ensure that these different events that happen during a cell cycle occur in the right order. OK, so this control system is going to ensure proper order. And there are two main components to this control system.
The first is called cyclin dependent kinase, or CDK. And so cyclin dependent kinase is a kinase, so it can post-translationally modify other proteins by adding a phosphate group to them. And so it's through that mechanism that cyclin dependent kinase can modify events and control when they happen in the cell cycle.
OK, and the other key component of the system is a protein called cyclin. And what cyclin is is it's the regulatory subunit of the CDK. So this is the regulatory subunit of CDK. And so without the cyclin, the cyclin dependent kinase is inactive, OK? So the CDK needs the cyclin to have activity. So cyclins increase the activity or activate CDK.
OK, but there are different flavors of cyclins. There are actually many different cyclins, at least four classes of cyclins. And these cyclins appear at different phases of the cell cycle and then go away. OK, so the cyclins oscillate that's why they're called cyclins, because they come on and off.
And depending on which cyclin is present determines what CDK is going to phosphorylate, OK? So these cyclins also determine substrate specificity of the kinase. So which cyclin determines what protein the CDK phosphorylates.
So I've outlined three classes of cyclins here. Here is a G1S cyclin in complex of CDK. And then here's an S cyclin complex with CDK in red. And so what do you think the S cyclin CDK is going to phosphorylate, what kind of protein? Anyone have a guess? Miles--
AUDIENCE: Helicase.
ADAM MARTIN: Yes, you're actually exactly right. It's going to phosphorylate and activate things that are involved in DNA replication. And Miles is right. Helicase is one of the proteins that gets phosphorylated by S cyclin CDK.
And then similarly, M cyclin CDK, which appears here in blue during mitosis, M cyclin CDK is going to phosphorylate proteins that are involved in forming the mitotic spindle so that it induces cell cycle events that happen specifically during mitosis. OK, so you all see it depends which cyclin is present that determines what cell cycle events are happening at a given time. And therefore, it's important that we understand how these cyclins appear at distinct cell cycle phases and whether or not that's the mechanism for the oscillation. Yes, miles--
AUDIENCE: I have a question about [INAUDIBLE] question about mitosis [INAUDIBLE]. So I know that microtubules make sure the chromosomes separate from the cell. How does a cell regulate having half of the organelles on each side [INAUDIBLE] split [INAUDIBLE].
ADAM MARTIN: Some organisms employee motors such that organelles are physically sort of put in daughter cells. But I think often it's just random, right? If you dissolve the organelle and it becomes kind of a bunch of different vesicles, then if you just split in half, there's a high probability that each daughter cell will get parts of the organelle, OK? So organelles can change their morphology during the division process in such a way that they're able to be inherited by both daughter cells. OK, that's an excellent question.
So it's the cyclins that really determine what's happening. And I just wanted to point out here that one of the main transcriptional targets of RTK signaling is this G1 cyclin, OK? So it's these signaling pathways that lead to the increase in G1 cyclin that start the cell on this process of entering into the cell cycle, OK?
So you get these cyclins getting synthesized. And the cyclins appear in a defined order. OK, so there are different cyclins, but they appear relative to each other in a stereotypical order. So they appear in order. And it's that order of the cyclin that defines which cell cycle events happen at what time in a cell.
OK, now I want to tell you a little bit about how the machinery that's involved in the control of the cell cycle was discovered. And I'm going to start by telling you a little bit about budding yeast. And by showing you how this was discovered, it will give you a sense as to how this system controls the cell division cycle. You'll recall that budding yeast can exist as a haploid cell in addition to existing as a diploid cell. So there's a haploid/diploid life cycle.
Also, one nice feature of budding yeast for this particular question is that you can infer the cell cycle morphology of the yeast just by looking at its morphology. So budding yeast divides by budding. And the size of the bud indicates what cell cycle phase the cell is in, OK? So you can infer cell cycle phase by morphology of the yeast cell.
So for example, if we look up here, here's an unbudded yeast cell that's probably in G1. Here's a yeast cell with a little teeny bud on it. That's probably an S phase.
Next to it over here is one that's a slightly bigger bud. And here's one with an even bigger bud. And that one might be in G2 phase. OK, so because this bud grows in size over the course of the cell cycle, you can just look at a yeast cell and infer what the cell cycle phase is.
OK, so I'm going to tell you about a genetic screen that was done to look for mutants that were defective in the cell division cycle. And these are known as cell division cycle, or CDC, mutants. Now, what type of yeast cell, haploid of diploid, might you want to screen mutants with? What would be the advantages of either one or the other? Yeah, Natalie?
AUDIENCE: Would you do haploid, because if it's a recessive mutation [INAUDIBLE] expressed?
ADAM MARTIN: Yes, so what Natalie suggested is to start with the haploid mutants because there's only one copy of each gene such that if you hit it, now you no longer have a functional copy of that gene. If you started with a diploid cell, you'd have two copies of the gene. And you'd have to have two mutations both happening in the same gene, which would be a rare event. OK, so it's better to start with a haploid in this case.
Now, what's the problem if you mutate a gene that's involved in the cell cycle? What's going to be the phenotype, the immediate visible phenotype? Is it going to be alive or dead? Carmen--
AUDIENCE: Dead.
ADAM MARTIN: It's going to be dead, right? And it's hard to work with an organism that's dead. OK, so what was done is to look for a particular type of mutant which is known as a temperature sensitive mutant. And a temperature sensitive mutant is a mutant where the cell or organism is alive and well at one temperature but dead at another temperature, OK?
And so the screen basically involved taking yeast. Here's yeast growing in a test tube. And this is now haploid yeast. And you can treat that yeast with a mutagen. It doesn't matter what, just something that will induce mutations at a high rate in these yeast cells.
And then you can take these cells and plate them on media where individual cells will grow into colonies. OK, and if you grow it at 22 degrees C for yeast, this is the most moderate temperature you can choose. So this is what's known as the permissive temperature. OK, but you can also take this plate of used colonies and duplicate it and grow it at another temperature.
And you might get something that looks like this, where you see this colony grew at 22 degrees, but at 37 degrees C it did not grow. And that would suggest that, then, this has a temperature sensitive mutant. And this temperature of 37 degrees is known as the restrictive temperature. OK, so that would identify a temperature sensitive mutant.
Now, is every temperature sensitive mutant that you identify, is that going to be a cell division cycle mutant? Miles, you're shaking your head no. Why is that? Can you explain your logic?
AUDIENCE: So there could be a couple different proteins [INAUDIBLE] there's too many mechanisms in the cell that could be dependent on temperature to narrow down to just [INAUDIBLE] mutant cell cycle. For example, if a phytoprotein mutant organism would mutate [INAUDIBLE] temperature sensitive, without that protein it would die also.
ADAM MARTIN: Exactly. So Miles is suggesting that if you just mutated any old gene that was involved in viability for this yeast, and it unfolded at 37 degrees because you sort of made a mutation that made it unstable, then you would identify that as a temperature sensitive mutant. So what would be a good criteria, I guess, that we could use to select just the mutants that are affecting the cell division cycle? Might there be a way for us to do that? I guess I'm asking, can we narrow down the phenotype, right? Temperature sensitivity could be-- by affecting any process in yeast, is there a way we can gear it towards the cell division cycle? Diana--
AUDIENCE: Maybe [INAUDIBLE] specific phase of the cell cycle, you could look at the morphology of it. And if all of them stop at the same phase, you might assume that you [INAUDIBLE].
ADAM MARTIN: All right, very good. So Diana is suggesting is that we looked for a phenotype. And she's guessed that the phenotype, if this gene is involved in sort of mediating a change from one cell cycle to phase to another, that if you mutate that, you'd have yeast that's all stuck in one phase, OK? And that's indeed the phenotype that was screened for.
OK, and so if you just take a random population of yeast that's dividing, you'll see cells that are unbudded, small budded, slightly bigger budded. And if you count the number of cells, what you'll see is that most of your cells are unbudded. Some are small budded. And a larger percentage are large budded.
And this just reflects the relative amount of time that yeast is in each of these phases of the cell cycle. So yeast spends most of its time in G1. Therefore, if you look at a random population of yeast, you'll see most of the cells will be in the unbudded state. OK, so this is for wild-type normal yeast.
Now, what was identified is a cell division cycle mutant, CDC 28, which at the restrictive temperature causes a train wreck at a specific phase of the cell cycle. So all of the cells now are stuck in the unbudded state. And so this suggests that these cells, when they are shifted to the restrictive temperature, are still able to move through the cell cycle. But once they get to this phase, they get stuck. OK, so here there is a cell cycle arrest at G1. And they confirmed it was G1 by measuring the DNA content.
And by measuring the DNA content, they were able to show that these cells did not duplicate their DNA. So they didn't even start to undergo S phase. They were stuck in G1, OK?
OK, so that suggests that the CDC 28 gene is required for cells to go from G1 to Sl. OK, so the wild-type CDC 28 gene is required for this transition from G1 into the S phase. And it turns out that this yeast CDC 28 gene is the one yeast cyclin dependent kinase, OK? So this was sort of the defining mutant for cyclin dependent kinase.
And you'll recall earlier in the semester when we talked about molecular biology that we talked about work done by Paul Nurse who used functional complementation to clone the human cyclin dependent kinase by transforming DNA into a different yeast, fission yeast. But again, that rescued the cell cycle arrest. And that's how the human cyclin dependent kinase was discovered.
And I just wanted to point out here that the work I'm telling you about was awarded the Nobel Prize in physiology and medicine in 2001. And it was awarded to Leland Hartwell, Tim Hunt, and Sir Paul Nurse. Leland Hartwell did the screen that I just told you about there and identified CDC 28. And we already talked about Paul Nurse earlier in the semester. Tim Hunt worked on clams and sea urchins and identified cyclin. So this was the work that identified the regulatory machinery of the cell cycle.
And they sort of showed that this worked in a number of different organisms. And they showed that it was evolutionarily conserved from yeast all the way to humans. OK, so this is a conserved mechanism.
OK, so there's a mechanism that actively governs the transition from G1 to S. And I'll point out this transition is known as start in yeast. And it's called the restriction point in mammalian cells.
It's kind of the point of no return in the cell cycle. But the cell cycle doesn't just blindly charge through the rest of the way. And there are certain quality control mechanisms that are in place to ensure that things happen in the proper order and that the quality of events happening is good before the cell moves on to the next stage.
And so I'm going to define a concept called a checkpoint. And the checkpoint is a type of quality control mechanism. And checkpoints operate in the cell cycle to ensure that one event doesn't occur till the preceding event happens correctly, OK? So this ensures proper order of events and ensures that events happen correctly before the next subsequent event has to occur.
OK, so one example of this is, if you just consider S phase and M phase, DNA replication has to finish before the cell starts segregating chromosomes. Otherwise there's going to be catastrophic consequences, such as possibly creating a cancer cell, OK?
So one example of a checkpoint is called the DNA damage checkpoint. And what the DNA damage checkpoint does is it looks to see if there's DNA damage or if the DNA is still replicating. And if either of these cases is present in a cell, then it sends a signal. And that signal, in order to influence the cell division cycle, has to interface with the cyclin CDK control machinery, OK? So this signal will then inhibit cyclin CDK.
And cyclin CDK governs two major transitions in the cell cycle. So there are two major what I'll call transition points. There's the G1 to S, which I just outlined over there, which is called start. So there's G1 to S. But there's also G2 to M, OK? So these, basically, the transition out of the gap phases, those are the key transition points that can be regulated by the cell to either slow things down to halt the transition or just go right through, OK?
So let me tell you about an experiment that defined the functionality of the DNA damage checkpoint. And I'm going to tell you about work done by Weinert and Leland Hartwell. And it was published in 1988. And they were interested in what the nature and the function of these checkpoints was.
And so you can take budding yeast. And you can damage its DNA by irradiating the cells with X-rays. And if you irradiate the cell with X-rays in a wild-type normal yeast, so in the normal yeast that's not mutant, then this damages the DNA. And the cell stays in G2. OK, so it stays in G2. OK, and there's a delay.
OK, so here what I'm drawing is a G2 delay. So the cell spends an abnormally longer time in G2 than it normally would if you didn't damage its DNA. All right, and then over time it will continue in the cell cycle and enter into the next cell cycle. And what's interesting about this is that these cells live.
OK, so one interpretation from this result is you damage the cell's DNA. It delayed the cell cycle in G2. So it didn't rush right into chromosome segregation. And that allowed the cell time to repair its DNA. And that enabled the cell daughters to live, OK? So that's an interpretation.
Now, part of the evidence for that interpretation is that Hartwell and Weinert discovered a mutant called RAD 9, so the RAD 9 mutant. And RAD 9 stands for radiation sensitive. This is a radiation sensitive mutant. And this particular radiation sensitive mutant disrupted the delay. So it disrupted the checkpoint here.
So what happens in a RAD 9 mutant is, again, you irradiate cells with X-rays. The cell goes from S phase to G2. But this time, there's no delay, so it goes from G2, the poor yeast charges unsuspectingly into mitosis with damaged DNA and divides. But in this case, there's a high level of death in the resulting progeny. So here you have death. OK, so RAD 9, then, is a gene that is involved in promoting the cell cycle delay such that the yeast cell has time to repair its DNA.
And if you remove that delay-- so here there's no delay. If you disrupt this delay, which defines the checkpoint process, right? The checkpoint is a process whereby if there's DNA damage, you delay the cell cycle such that the cell has time to repair it. If you don't have that, it has bad consequences for the cell and results in your cells undergoing premature mitosis before they've had a chance to repair the DNA.
Let's see. I'm going to use this one here.
All right, one thing you might be wondering is what causes these cyclin proteins to oscillate. And so the last point I want to make is I want to tell you about the mechanism that allows these protein oscillations. And it involves a mechanism that's going to be new for you, at least from the context of this class. It's a mechanism that's regulated proteolysis.
OK, so there's a regulated degradation of these cyclin proteins that allows them to go up and then down, OK? So if we consider just one part of the cell cycle, well, if I plot the concentration of cyclin and we look at M cyclin from G2 to M phase-- so this here is a time axis-- the M cyclin goes up in M phase. And then it drops precipitously during mitosis, specifically at the metaphase/anaphase OK, so this is for the mitotic cyclin. And I'm going to tell you that this precipitous decline in cyclin levels is due to regulated proteolysis.
OK, and it involves a mechanism that you were briefly introduced to by Professor Imperiali, because it involves a small protein known as ubiquitin. What ubiquitin is is it's a small 76 amino acid protein. So it's a 76 amino acid protein.
But this protein can get attached to other proteins. So it's a post-translational modification. OK, so ubiquitin, which I'll abbreviate UB, ubiquitin is attached to lysines on a target protein. And the attachment of ubiquitins to lysines of a protein has important consequences.
And what Professor Imperiali told you about is when this happens in the case of protein misfolding. But this ubiquitination of proteins also occurs to proteins that are not denatured or misfolded. And it's a way of regulating protein levels in the cell.
OK, so what happens is-- I'll show you a complicated diagram of what happens. But I'm really going to focus on this step right here. There's a series of steps that are needed to get ubiquitin to get attached to the target protein. I'm going to ignore pretty much all of that.
But there is an E2 enzyme that becomes conjugated with ubiquitin. And then the ubiquitin is able to be transferred to a target protein. And rather than make this generic, I'll let you know the target protein is going to be cyclin. So we'll just say cyclin.
And so there's an enzyme that transfers the ubiquitin from the E2 to the cyclin, OK? And it's polyubiquitinated, meaning there's a chain of ubiquitins added to the cyclin. And it's carried out by a particular type of enzyme known as an E3 ubiquitin ligase.
And there are hundreds of these ubiquitin ligases in humans. And different E3 ubiquitin ligases confer different specificities. So they target different proteins, OK? So different E3's target different proteins. And so this is where the specificity comes from, OK?
So when a protein in the cell, misfolded or not, is polyubiquitinated like this, this is a garbage tag on that protein, OK? So you can think of polyubiquitin as a garbage tag. And once it's polyubiquitinated, it's sent to the proteasome, which Professor Imperiali showed you. And this is the structure that degrades proteins. OK, so if the protein is targeted for degradation by putting this tag on it, it's going to be rapidly proteolyzed in the cytoplasm of the cell.
All right, now I want to show you some experiments that provided the first evidence that this regulated proteolysis is what sort of causes the cyclin to oscillate, OK? And it's going to involve a new model organism. Is there anyone here that has ranidaphobia? OK, we all like frogs?
OK, so this model organism is xenopus laevis, or the African clawed frog. And I want to thank my colleague at UMass Amherst, Tom [? Oreska, ?] who provided the slides of frogs for me. So what's great about these frogs, well, other than them being very cute, is that they lay a ton of eggs. And the eggs are huge, OK?
So here is a mom, a mom frog. And then you see all these circular things all around the frog are the eggs. OK, so they're about 1 millimeter in size. They're huge. You can collect these eggs, put them in a test tube. And you can see all these eggs that you have in this test tube. And then you can spin the test tube.
And by spinning the test tube in a centrifuge, you crush the eggs. And so that results in this middle layer here, which is cytoplasm, OK? And you can remove it with a syringe. And what you end up with is a concentrated cytoplasmic extract. So that's all cytoplasm. OK, so that's a lot of cytoplasm.
OK, so for the xenopus system, this system allows you to get this highly concentrated-- because it's not diluted. It's the same concentration as cytoplasm almost-- cytoplasmic extract, which is known as xenopus egg extract. OK, and what's amazing about this egg extract is it can go through the cell cycle even though it's not in a cell. OK, so you can get this extract to essentially simulate the cell cycle.
OK, so you have to mimic fertilization, because that's when cell divisions start to happen in the normal frog embryo. But once you do this, then you mimic the fertilization process by adding calcium, and then you'll see this extract go through the cell cycle. And you can see it by looking at the morphology of different structures in the extract.
So this is a nucleus that has assembled an extract around some DNA that was added. OK, DNA replication can happen in this nucleus. Other events that happen in the interphase of the cell cycle also happen in this extract. And if you wait, then it will go into mitosis. And you'll start to see mitotic spindles assembling in the extract.
OK, so what's important about this, this is totally in vitro. OK, so this is an in vitro system. There are no cells. But you're able to see the extract go through the cell cycle. OK, and if you were able to look at cyclin, like M cyclin, you'd see that M cyclin levels go up and then down and up and down. They oscillate just like they would in a cell.
And this is just a diagram showing you that here where mitotic cyclin, M cyclin, concentration is in blue and CDK activity for M cyclin CDK is in purple. So you see it goes up. And then the cell enters mitosis.
Early mitosis is in blue. Late mitosis is an orange. So you see mitosis happens when M cyclin is high, just like it does in a cell. And then it degrades. And then it repeats.
OK, so this is all outside of a cell. But you're just looking in a test tube. And you can recreate the cell cycle.
Now, this, because this is a biochemical system, allowed these researchers-- in this case, the researchers who did this experiment were Andrew Murray and Mark Kirchner at Harvard. And what they did was to test the role of various components in this oscillation. The first experiment they did was to RNase treat the extract to get rid of all the mRNA.
And if you degrade all of the mRNA in this extract, you no longer get the cycling. You no longer get the cell cycle. So this shows you mRNA is important or necessary. But you don't know which mRNA, right? One hypothesis might be that you need the mRNA from M cyclin in order to produce cyclin every cell cycle. And that was their hypothesis.
So what they did to test that was to degrade all the mRNA, inactivate the RNase, and then add back the mRNA to one gene, that mitotic cyclin. And what they saw when they did that is they restored the cell cycle, suggesting that this one mRNA, M cyclin, is sufficient to restore the oscillation of the mitotic cycle.
OK, now, the last experiment, which I think is the most important, shows you the mechanism by which the cyclin is going. Because they added-- and instead of adding back the wild-type mitotic cyclin, they added back a cyclin mutant that was non-degraded all by this E3 ubiquitin ligase mechanism. OK, so if they add back a cyclin mutant and this mutant has a deletion in the part of the protein called the destruction box-- and this is essentially the part of the protein that is recognized by the E3 ubiquitin ligase, OK? So the destruction box mutant basically blocks this such that cyclin is no longer polyubiquitinated and it can't be targeted for proteolysis.
OK, and in this case, what happens is cyclin levels increase. And then they stay high and there's no cycle. OK, so when you have this cyclin mutant with the destruction box deleted such that it's non-degraded, it's not degraded, you get a cell cycle arrest. And because this is M cyclin, the cell arrests in mitosis. You get a mitotic arrest, a mitotic arrest.
OK, any questions about this mechanism of proteolytic degradation? You all see how this-- yes, Malik?
AUDIENCE: So [INAUDIBLE] cell [INAUDIBLE] what is it physically doing?
ADAM MARTIN: What is it physically doing? It's basically stuck with a mitotic spindle and it's not segregating the chromosomes. Yeah, so it hasn't gone through mitosis. It's stuck in a specific phase of mitosis. In this case, it's stuck in basically a metaphase-like state.
One last point I want to make about this is that the mRNA for M cyclin is just constant. It's always present. So this is constant. You have constant mRNA. CDK is constant. It's the cyclin protein that's going up and down. And it's going up and down because of this regulated proteolysis.
OK, great. On Wednesday, we'll talk about stem cells and we'll talk about guts.