Flash and JavaScript are required for this feature.
Download the video from Internet Archive.
Description
Professor Imperiali goes through the mechanisms of how bacteria develop resistance against antibiotics. Following this, she introduces viruses and discusses some of their characteristics.
Instructor: Barbara Imperiali
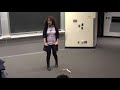
Lecture 33: Bacteria and An...
BARBARA IMPERIALI: OK, I want to walk us through a bit of an exercise to understand what happens when people become resistant to an antibiotic. What's the molecular basis for resistance? It's nothing magical. It's really things that you can understand based on what you've learned during various parts of the course. But I just want to remind you about this dreadful schematic here, which shows how rapidly resistance emerges to different antibiotics by showing you the year that the drugs are introduced and the year that resistances develop.
One of the newest antibiotics to be introduced-- people thought, oh, it's a different mechanism of action. It should be pretty resilient. It should last for a while. It should be useful with daptomycin. It's a cyclic peptide antibiotic, which has a particular structure that doesn't look like a lot of the others. And honestly, it was two to three years before resistance emerged. So what I want to do is think together about what are the ways in which a bacterium could evolve to develop resistance against an antibiotic?
So here we've got the target. We know that the antibiotic is very effective against the target. What types of things could happen in the bacterium to make it manage to just ignore the antibiotic and resist the antibiotic? Any suggestions? So it's very simple. There's a molecular target. It could be topoisomerase. It could be in fact the ribosome and the machinery for synthesizing proteins. It could be the machinery that cross-links peptidoglycan. What sorts of approaches and what sorts of strategies might evolve to make that antibiotic stop working? OK, fire. Yes.
AUDIENCE: When two hydrogen-holding enzymes at-- create things that are not [INAUDIBLE] absorbing.
BARBARA IMPERIALI: OK, right. So antibiotic gets in. The enzyme breaks up the antibiotic, so evolution to destroy the antibiotic. And that's very much what happens with penicillin. The key aspect of the structure that's so useful suddenly becomes invalidated through a degradation of the beta-lactam bond. So that's one of them. What's next? Yeah.
AUDIENCE: [INAUDIBLE]
BARBARA IMPERIALI: Ah, so maybe there could be uptake decreased, so that's quite hard, but there could be some evolution of the cell wall to make it less permeable to the antibiotic because we're usually relying on antibiotics to diffuse in passively. So if the membrane-- this could be in a membrane of a Gram-negative bacterium, or the outer membrane of a Gram-negative bacterium that has a slightly different composition, could physically change its structure. These are tough things to evolve all at once, but it's certainly a possibility. We've talked about uptake. What about-- what else could happen? Yes.
AUDIENCE: When you get a [INAUDIBLE] out, such that they have oxygen [INAUDIBLE].
BARBARA IMPERIALI: OK. So this guy could just-- we've got a circular antibiotic usually, but this just changes-- the target just changes. It can't bind anymore, through mutation, so that the antibiotic simply doesn't bind. It gets in, but it's changed, and that happens a lot.
It also happens a lot-- one can think of antibiotic resistance very much along the same veins as one thinks of resistance to chemotherapeutic agents. You're targeting a kinase. Your drug works great. A year later, the cancer comes back because there's a single mutation in your target. This happens a lot with the EGF, kinase, RAS's and so on. There's a dramatic change. So this change would be a change in the target. You mentioned two things, right? I thought. Maybe not.
AUDIENCE: Well, like I said, there were some for that [INAUDIBLE].
BARBARA IMPERIALI: Ah. On the surface.
AUDIENCE: A couple of days you're going back, so.
BARBARA IMPERIALI: OK, so there could be some kind of import strategy. So some antibiotics just diffuse. Some go in through targeted import, and that might change so that the antibiotic can't get in anymore. Other thoughts? There's a couple of other sneaky ways. I mean, you've got to sort of give these bacteria credit for maximum sneakiness.
So if influx is an issue, what about efflux? The biggest problem with antibiotics against Gram-negatives is that they way upregulate efflux pumps. They just, like, cover their cells with pumps that just go, you're going to give me an antibiotic? I'm just going to lob it straight back out to you. So the efflux pumps increase. So their molecules, we have a lot of efflux pumps to just kick out things that are not-- that we don't want in our cells.
The bacteria have similar things. They will-- they're fairly promiscuous, exporting pumps that will bind to things that don't look like things that should be in a cell, and literally bind to them on the inside of the membrane, [MAKES SQUIRT SOUND] send them back to the outside of the membrane.
So Gram-negative bacteria can massively upregulate the production of a pump that they already have, but they just make much more of them. So in many cases, you can hardly test a new compound because it's getting pumped out as fast as it gets pumped in.
What could be a strategy when this happens? Because people are doing this. You inhibit the pump. So you make your antibiotic-- it works great, but you stop the pump working. So you have to give two drugs, the drug that's the antibiotic to the target and the drug that inhibits the pump, and that happens.
Similarly, with this mechanism, where the antibiotic gets destroyed, you can recover from this by inhibiting the destroying enzyme, and then your antibiotic doesn't get destroyed when it gets into the cells. So there's one extremely important formulation of antibiotic that is used. It's called Augmentin.
And what it is is a penicillin plus a beta-lactamase inhibitor. It's the drug that works plus a drug that inhibits the enzyme that destroys the drug. People get this all the time. Every day of the week, this stuff is prescribed. It's a two-compound cocktail that has something to overcome the resistance so that your drug still works in a cell. All right?
And there's one more key mechanism, and what bacteria will just do is they say, well, you know, I'm getting dosed with this much antibiotic. What's a good way around it is to massively upregulate the biosynthesis of the target to a state where you just can't saturate it all. So upregulation of the target is a very, very common thing. So you-- this should have just increased the number of transcripts being made. You increase the amount of target being made so that, even if antibiotics are flooding into cells, there's just not enough to inhibit all of the target because it's been upregulated by 10 or 100-fold.
So what I think is cool about all these mechanisms is they all make sense. You just kind of have to think of them-- do I stop the drug getting in? Do I stop it getting pumped out? Do I stop it getting degraded? Do I make more target? All of those things are very viable, and they are strategies that are used quite commonly.
So very commonly, both in bacteria and in viruses, we seldom give one compound. We commonly give multiple compounds to sort of hit multiple targets, because if you gave a two-drug cocktail to a bacterium, but you knew there was going to be upregulation of a target, you could hope that the other enzyme is still a target. So you give cocktails of drugs, as opposed to single drugs.
And you're going to see that very relevantly when we talk about the HIV virus because it's only been that HIV has become-- HIV-AIDS has become a treatable condition because of drug cocktails, not because of singular drugs that inhibit one step. And you'll see it very, very commonly there. Any questions about this stuff? OK.
So we're going to move on to viruses. And so I will actually update the slides that are on the web to give you this set of information so you can see it in one place. OK, viruses. Viruses are fascinating organisms. They don't have the right to be alive because they don't have the machinery to be alive, but they exploit the host's mechanisms for completing their viability. So viruses, more or less, I think we like to think of them as living a borrowed life. And they only survive if they have spent some time inside a host cell.
So we will see with viruses, some viruses specifically target humans. Other viruses may spend some time in different organisms, and then target humans, and be carried around amongst different organisms. But the key thing is that viruses can only actually replicate once they're inside a host cell because they basically exploit all of the host cell's machinery to do that.
So viruses don't make many of their own enzymes. They don't have their own amino acid supplies or all the metabolic enzymes that are required for life, all the replication, transcription, translation machinery. They just borrow the host's machinery, but there are occasionally individual components that the virus will bring along with it to cover certain things that are not provided by the hosts.
But viral genomes are tiny. They may comprise maybe eight genes. Some of them are a lot-- are bigger, but they are very, very small genomes. They're very, what we call, parsimonious genomes, so there's overlapping genes, so you can keep the genome tiny by having-- compacting the size of the genome.
And then there are bigger viruses. Some of the biggest viruses approach the sizes of bacteria, like the mimiviruses, and they may have been an intermediate step from virus to more elaborated organisms. And those viruses have a bit more machinery within their contexts.
People-- obviously, there's no fossil record for viruses. It's not like we can go, you know, go exploring and find a fossil record. But where these viruses are being found is in the permafrost, so they're frozen. They've been frozen there for centuries. So people are finding really sort of scary things in the Siberian permafrost because the viruses are preserved there. And some of these giant viruses are being discovered in those locations.
And if that's not the subject for wonderful sci-fi books, I don't know what is, because there are-- and I tend to read those things because my favorite thing is finding mistakes in them. So there's a lot out there about those kinds of things.
So let me just show you a tiny bit about-- you know, this is that boring old "learn genetics" thing right from the beginning, but what I want to take you back to is sizes. So we know all this stuff. We've learned it to death, hemoglobin, antibodies, and ribosome. But what I really want to point out on this slide is that the smallest viruses, like the rhinovirus-- that's the common cold-- or the hepatitis virus are not barely any bigger than the ribosome.
So obviously, there's not much in the virus, but all the components of the virus have evolved to enable them to sneakily get into host cells, exploit the host cell machinery, and then replicate inside host cell, and then get out of the host cell and ready to infect another host cell.
So I want you to really notice these sizes. So the rhinovirus is similar to a ribosome, but some viruses, which we will talk about, the influenza virus and the HIV virus are a little bigger, but none of them compete up to the size of a bacterium. And just to get you into that mode, there are the bacteria, and there's the mitochondria, and remember endosymbiotic theory, bacteria, similar size to mitochondria, and much, much bigger than any typical virus.
But giant viruses approach some of these bigger sizes. They're a different ballgame altogether. And we won't talk about them, apart from the fact that they're really cool, and they're in the permafrost.
All right, so another impressive thing about viruses, as they look-- some of them like this. Phage, a bacterial virus, they look like things, lunar landers, for example, or sort of other kinds of things. Some of them are linear. Some of them are different kinds of shapes. A lot of viruses are icosahedra. We'll talk about that in a moment.
But the fact sheet about viruses is first of all sizes. And the typical viral size, if there is something typical, expand from 20 to 400 nanometers in diameter. So remember, the ribosome sits right at this end with respect to size, but bacteria-- oh, yellow, I can't do a yellow lecture here-- remember, are 1 to 10 micrometers in length, depending on what dimension you're measuring, so considerably smaller, nanometer scale, micrometer scale for bacteria. So that's the first thing that it's important to know. They're very small.
The next critical thing is what's in a virus? What is its-- what's the blood and guts of a virus? And it's either DNA or RNA, and it can be single-stranded or double-stranded. So it has its genetic material. Its genetic material is usually dedicated to making more copies of itself.
So if the virus has a coat, a coat of proteins, the virus has to have a gene for that because the host isn't going to have a coat for a virus. So the virus has to have certain specialized things that complete itself that can't be borrowed from the host cell.
And they can be-- let's see-- they can be what are called capsid viruses or enveloped. Capsid viruses just have a protein coat. The enveloped viruses have a membrane surrounding them with proteins stuck into them. So the enveloped viruses have an outer membrane which is studded with proteins.
But what is cool about the virus is it never makes its own membranes. It doesn't make its phospholipids. It just, as it emerges from a host cell, pinches a piece of the cell surface. It steals the cellular membrane with it, as it's emerging from a cell. And you'll see this in a video, how cool that is, all the proteins that-- all the components of the virus cluster near the surface of a membrane, and then you have this wonderful endocytosis using the host membrane. So the virus never has to make it own membrane. And not all viruses are enveloped, just some of them, and you'll see examples of each.
So the definition really is that they're small, infectious agents, that they only replicate inside living cells because they have to exploit a lot of the machinery of living cells. And they can infect humans, other animals. There are plant viruses. Bacteria have viruses. So all living organisms have viruses that infect them.
But viruses are usually targeted very specifically to the cells that they infect. And, in fact, you will see with HIV, it's not just a virus that infects a human host. It infects specifically, and that was why it was so terrifying, this-- actually T cells. That's the one cell type in the host that it goes after. So viruses very often target to particular organs within their hosts, and that's why we know some of the viruses. And you'll see that the names of the viruses are related to the organs that they may infect.
So I want to just briefly describe the terms. We talk about these with all infectious diseases, that they may be endemic, epidemic, and pandemic. Endemic is the term that we use for, there's a very low level of an infectious agent in the population. It's completely out of-- within control. There's a few cases, but there's not a transfer from person to person or animal to animal. We would call that endemic, a very, very low level of virus that doesn't cause any threat.
As soon as the virus or bacterial infectious agents starts spreading amongst a local population, we would call that a local epidemic, so all of a community, all of a country, so very much defined geographically into a particular space where there's transmission of viruses from person to person or animal to person within a community. There has to be direct contact.
But now, with travel, many viruses reach pandemic stages, which means worldwide. So plane travel really caused enormous trouble because you can have a virus in Africa or Asia. Somebody gets on a plane and ends up somewhere else, and the virus has been moved to a new country.
I made the terrible mistake of reading Hot Zone on a plane one time, which is about Marburg virus, which is where people basically just start bleeding out in the spot. And I'm reading this book, and it's describing the steps of someone who had Marburg and was just sort of bleeding out next to them on the plane. And I'm like, are you crazy, reading this book on a plane? Because they were describing how Marburg was just moved from its country of origin to New York City, or something like that.
So you remember when we had the Ebola concerns. There was a real, genuine worry that Ebola would jump through flight travel, through people coming in at airports, and end up with a pandemic of Ebola.
When there was a real problem with the avian flu in Asia, Singapore, that's very, very protective of its territory, had sensors that would-- you would go into Singapore, and you'd go down these two huge escalators. And they had sensors measuring people's temperature at a distance as they came down the escalator, and hauling people over, and sort of interrogating them, where have you been?-- to see whether they would be allowed to enter Singapore, because the flight travel, people getting on planes, spreading a very contagious virus to a new country is very, very realistic.
The issue with spreading to pandemic situations is very, very important when one thinks of history, because when the Europeans were conquering the Americas, in particular South America and Central America, they brought with them a lot of viruses. But there was an innate sort of resistance to-- because of years and years of exposure.
But these communities have never seen these viruses, so millions of people died because they were suddenly exposed to a human virus that they had never seen before, through transmission from a country where there wasn't such a problem with the virus. So the indigenous peoples of the Americas, Australia, and New Zealand had terrible consequences there.
Some of you have probably heard of the Spanish flu, and that was towards the tail end of World War I and is thought to have killed as many as 100 million people. And that, in fact, is quite interesting. It's called the Spanish flu, but there's some evidence that it might have originated in the Americas, in the boats that took troops over to Europe to help at the tail end of the First World War. And there's a really interesting book about that whole story, that the Spanish flu may not have originated in Spain. And that's a-- it's definitely a worthwhile read.
So that tells you a lot about the statistics of viruses. I just want to highlight here, we talk about HIV as a very serious virus. It emerged in the early '80s to this current-- well, in 2011, there were 35 million people infected. There's about 2 and 1/2 million new cases a year.
But what's fascinating about HIV-- there was a stage before the really good antivirals were available that, if the mother had HIV, the baby would get HIV. But now, if there's treatment of the mother, and the baby is delivered, often by a Caesarean section, the baby can escape being infected with the virus due to the new antivirals.
So that's really important, that the-- originally, there were a lot of cases of newborns who simply got HIV during birth. But now that can be-- there's escape from that, which is really, really cool. The viral load can be brought really low with the common antivirals against HIV, and that next generation doesn't have that sentence.
So I mentioned to you that a lot of viruses are basically named after the organs that they hit. So I've just got a human being here with a lot of different viruses that hit different places, and I just want to point out a few points. Viruses may be targets nowadays of childhood vaccinations, and many of you-- I hope all of you-- have had vaccinations to many of these common viruses.
There is a concern now with communities that are deciding not to vaccinate children. That's a huge social problem that may, initially, sort of, people can get away with it because there's community vaccination. You're in a community where a lot of people have a resistance or some sort of immunity to a virus. But as communities become less and less vaccinated, than later generations will start to get the disease seriously.
And that's actually happening in parts of the world where there's-- there used to be no polio, and now there's polio emerging because the community immunity has been-- is fading away. So we hope that people get vaccinated. That's for sure. The vaccinations work.
Several vaccinations-- several viruses were pretty much eradicated. Smallpox and polio were two of the real poster examples of childhood vaccinations that worked and worked amazingly well. But now, there's a problem with failure to vaccinate in certain parts of the world. So that's a concern.
And then, another interesting thing is that some viruses-- whoops, oops, go back. Sorry. Go back, back, back, back. Don't now-- that's all a secret. You can't see that just yet. Some viruses lead to cancer, so human papilloma virus, where there is a vaccine, the people who have HIV-- some of the types of hepatitis and Epstein-Barr are all associated with later cases of cancer. That's important to know.
So often cancers are named by the organ that they attach, so even though the three-- the five hepatitises all attack the liver, they're not related. They're just five viruses that go off to the liver. So if you've had a vaccination against Hep A, It doesn't protect you from Hep B or C by a relationship. They're very, very different, so you have to have different vaccinations.
So this just gives you a nice view of human viruses, what their names are, what organs they may attack, what sorts of things they might be associated with. But the trouble is, this nomenclature doesn't get you anywhere towards understanding the mechanism of a virus. So what we will focus on is a much better system for describing viruses that's based on whether they have DNA or RNA within the genomes that they import into host cells, and whether that DNA or RNA is single-stranded or double-stranded, because that truly tells us a lot more about the virus and maybe the steps that could be inhibited to prevent the viral infections.
But first of all, just a few pictures-- here's some-- so viruses can be rod-shaped. They can look like-- they can be icosahedra. They can just have a capsid. So I mentioned they may just have a protein coat, the sets of repeating proteins that pack into a beautiful structure, very commonly an icosahedron, and I'll show you why that is.
Or they may be enveloped viruses, like, influenza has a membranous surface around where the DNA is packaged. All of these have nucleic acids packaged within them, DNA or RNA, single or double-stranded. And in the case of the enveloped viruses, that membrane-- it's a normal membrane. It's just like your membrane. In fact, it is your membrane-- will have proteins dotted within it that's actually-- serve as recognition to the host cells. They'll grab onto host cells and be the source of the infection into the host cells.
And this is a bacterial virus, and as I said, I just love the way they-- I mean, they really look like this. You know, the cartoon is really the cartoon of what the thing looks like, and they're sort of pretty amazing. And they kind of-- they keep their nucleic acid in the head here. They land on their sort of feet, and they shoot the nucleic acid material into the host cells. So that's very interesting. Of course, this thing is-- OK.
So why are many viruses that are capsid viruses icosahedra? So it ends up being a problem of geometry. So how can you make a perfect coat around something with very, very few building blocks of different types? Like, if every building block in that coat was different, the virus would have to have genes for all of them.
What viruses can do is they can have genes for, like, three pieces of a module of the virus. So I'm going to show you how these capsid viruses get assembled. So here, color-coded, is an icosahedral virus, where I've coded in the red, green, and blue, a triangular component-- this is really cool-- that is a single sort of panel on that icosahedral virus that comes together as a triangle through noncovalent interactions between three proteins.
You see that panel there. What you can then do is see how that panel would fit into a pentagon with an extra triangle stuck onto it, and you can fit that triangle into the pentagon and also into the additional piece. And then you can start to visualize how you could build an icosahedron from those pieces because they represent each of those faces within the virus.
So you can go from this, which is a set of building blocks that I just showed you-- then you can assemble them like this. And one of these would be this part of the icosahedron, and then you just have a bunch of copies of it. And you can see how you would assemble that.
And years ago, I decided to decorate my Christmas tree with icosahedra. So I went through this geometrical thing, and believe me, it works really nicely. You can put together an icosahedron and build an icosahedron. You could spray it gold and put it on your Christmas tree. It's kind of fanatical, but it really-- it's highly recommended.
All right, so let's now get down to something a little bit more serious than Christmas trees and things. All right, so I told you that the classification of viruses by what organ they attack or who discovered them or anything is just-- is a vagary that's not so useful to the non-physicians because you can't immediately know, oh, this is how the virus gets into the host cell. This is how the virus uses its genetic material to make new viruses.
So what was developed by Baltimore-- David Baltimore used to be at-- it was kind of interesting. David Baltimore, a very famous person and Nobel laureate, used to be at MIT when I was at Caltech, and we moved in opposite directions. I'm not sure it was a great trade for MIT, but it was a great trade for Caltech. So I ended up with David Baltimore's labs in Building 68 because we did that swap in 1999 or something like that. So I thought that was pretty interesting.
Anyway, so what Baltimore decided is-- was much better to classify viruses by the type of genetic material, like, are they DNA or RNA? Is that DNA or RNA single-stranded or double-stranded? Because, depending on what the genetic material in the virus is, once that gets unloaded into a host cell, certain steps have to happen in order for the virus to be able to replicate that genetic material, to convert it ultimately into the proteins it needs, and then to package up new viral genetic material into viral capsids so that they can then be sprung out of the cell and go infect another cell.
So the classification basically went this way. So if you think of it, what the major goal in the infected cell is to get the virus to a stage where the virus has plus-messenger RNA. It has RNA that can be read by the host's ribosomes and convert it into proteins. So the overall goal of the virus, if we give it sort of some conscience, shall we say, is to make its viral material into messenger RNA.
Now, the virus doesn't include messenger RNA. That's just what is made transiently, but the virus may have single-stranded DNA. It may have plus-sense RNA. It may have negative-sense RNA. It could have double-stranded DNA, or it could even have double-stranded RNA. And depending on what that genetic material, is what the Baltimore classification of a virus would be. So depending on what's inside the virus, then they can be classified.
And what we're going to go through today and on Friday is examples of class I, class V, and class VI viruses, so we can see how that genetic material ultimately becomes a new viral-- a new virus within a host cell, or at least the components thereof ready to be sprung out of a virus.
And there's one important point that I want to also address-- oops-- budding or lytic. All right, there are two ways in which viruses escape their host cell. They may be budding. So here you have a host cell. The viral components all congregate near the surface of the membrane from the inside, and then the host cell buds off. The viral components go with it, and the bud splits off.
So the host cell has its nucleus. It's still intact. HIV is such a virus. HIV doesn't kill its hosts. That's the best sign of a parasite. It wants the host to stick around, so it just buds off.
The other types of viruses are lytic, and, basically, the cell just bursts open and throws out the virus. So they're in two categories. Some of them are budding, though the enveloped viruses have to be budding because they're going to take with them the membrane of the host cell. All right? So that's another important difference.
So what have we got here? So ultimately, the goal is to be able to make a plus-strand mRNA for protein synthesis. So we really need to have the appropriate sense of the RNA that will dictate the protein synthesis. So let's first of all look at one of the simple versions, a double-stranded DNA virus.
And this is represented by the smallpox virus. So everybody's heard of that, and herpes simplex. And these are both enveloped viruses, so that means they have a membrane shell. And so I'm just going to walk you through the steps of going from the double-stranded DNA to make a new virus.
So here's the virus. It has a capsid, as well as a membrane envelope. And there's recognition between the virus and the host cell. And we'll talk very specifically about what that recognition is when we talk about HIV, because that's very well categorized.
Once the virus gets into the host cell, it sort of spills off all the coat and dumps out its double-stranded DNA, the viral DNA, into the host cell. And then that DNA can-- in the nucleus, can replicate into more copies of the viral DNA, or it can be transcribed into messenger RNA, which is then the coat for all those capsid proteins that the virus needs.
And then these start to self-assemble within the host cell where the capsid proteins wrap around the viral genetic material. They accumulate near the surface of the cell, and then they bud off from the cell. So that's how you go from simple DNA.
So these processes are completely based on the human enzymes that do those processes. Replication, we've got to replicate DNA. We're going to have to do that in the nucleus. Transcription, we're going to have to ship out part of the DNA from the nucleus to the cytoplasm and make-- we're going to have to make a copy of the messenger RNA and ship it out to the nucleus.
And then we're going to use the host ribosomes, the host's amino acids, the building blocks in everything, to make a new protein that is not a host's cell protein. It's the capsid protein. Obviously, the human cell isn't going to be making a capsid protein. So that's the main thing that the virus had to encode. It had to have the DNA to make that.
So that all looks sort of fairly simple, and the steps make sense. This is why we cover this virus first because it really-- it's kind of the most transparent to understand, so this transient stage of sort of borrowing machinery. And as I mentioned here, the virus can spring out of the host cell. Yes.
AUDIENCE: So all it means is-- one's that don't kill the host cells, how do they, like, on the body, or the cells that they--
BARBARA IMPERIALI: They start to just be too much of a burden onto the body, so they're just-- you know, if they're inside cells and exploiting the resources of the cell, they're basically-- they're harming it, but they're not destroying it instantly every life cycle. They're just using resources to replicate, and then go-- get spread to another cell, and another cell, where they'll keep using resources. So it's really just an overload of the system. It's a very good point.
But they can stick around a long time, and with HIV, you're going to see what really sneaky thing they do is because they put their genome into the host genome. And that's sort of really pretty terrifying. I always ask this question, but it's kind of a silly one. You know, what is life? A virus is alive. Well, they're kind of alive, but they're not really alive unless they have some place to live. But aren't we all like that? So that's very philosophical. So we'll move right on here.
So when you think of a virus, this is the original central dogma, all the moving parts of the central dogma. And note-- so that when you think of a virus, what double-- what does double-stranded DNA need from the host? It's got all of these things. It's got the-- the host has the polymerase. It has the DNA-dependent RNA polymerase. It's got all the ribosomal machinery. So the only thing that the virus needs is the gene for its capsid proteins. So you can peel out from that entire life cycle the one unique thing about the virus. So that's a double-stranded DNA.
Let's now move to a different type of V, which is a negative-stranded RNA virus. And these are quite important because these form the basis for-- let me just go to the diseases. This is the influenza virus, and I'm going to mention some very important points relative to influenza virus.
So influenza virus is what's known as a segmented virus. And what that means is that its genome-- in this case, it's negative-stranded RNA-- is in pieces. A lot of other viruses just have a single strand of genome, a single nucleic acid strand. So it's just one piece, where portions of that nucleic acid code for different proteins, and they'll often code for initially polyproteins that get broken up. And we'll see a virus with a single strand when we look at HIV.
But the influenza virus has a segmented genome. And that's very relevant for its lifestyle because we'll see in a moment how influenza virus can cause more damage than we anticipate because of recombination of different copies of the segmented virus through differences.
But let's first of all take a look at the life cycle of this virus, and then we'll move on to dealing with the issue of the segmentation. So here's a typical enveloped virus with a capsid. Inside, there's the negative-stranded RNA that gets into the host cell, and you make-- and you dump into the host cell the viral genomic RNA. That can get copied. The minus-strand RNA gets copied to the plus-strand RNA, which becomes the messenger for protein synthesis in the cell.
So you've gone in with minus strand. You've made the plus strand, which is the messenger, and that encodes all the proteins that are needed for a new virus. And some of those proteins may have signal sequences. They may be shipped to the surface of the cell, and they may be planted in the outside cellular membrane of the host cells.
And what you see here is copies of those proteins actually in the surface of a cell. So what happens with this virus is, once all the moving parts are made, they congregate at the surface of a cell, get packaged, and then bud off from the cell.
So remember all the rules you learned about where proteins end up in the cell are all good still here because the capsid proteins have to get to a cell membrane, so they're translated with a signal sequence. They congregate-- I don't know how this self-assembly occurs, but it's a fascinating process, so that ultimately you bud off an intact virion from the host cell.
But the key thing that the virus has to have is something that will copy negative-stranded RNA to plus-stranded RNA, which is going to be the messenger. So the virus also has to code for a particular protein that's unique to its lifestyle. So it has an RA-dependent RNA polymerase.
We don't use an RNA-dependent RNA polymerase, but the virus needs it to take its negative-strand RNA to a plus-strand RNA, which will be the messenger. So does that make sense? So obviously, that's a moving part that it needs to provide to the host.
Now, what's this about segmented viruses that's quite important? Oh, and I just want to underscore here, what defines the destination of these proteins, whether they're capsid proteins or proteins that are going to be packaged within the virus, is basically just the same rules that apply that we talked about when we talked about protein trafficking.
So every year, there's a whole panic. Did you get your flu shot? Is it going to work this year? Oh my god, millions of people are going to get sick. Go get your flu shot. It's tetravalent, it's trivalent, and so on. So what we're trying to do every year is predict what the virus is going to look like.
So we have to-- there are teams of people, who sometimes get it wrong, who predict the variation in these genes. And they look at winter in the Southern Hemisphere, because that precedes us, and try to guess what's going to happen in winter in the Northern Hemisphere. And we get to try and put together a vaccination package.
But the problem with the viral influenza virus is that there can be not just a drift, like mutations, small mutations happening a little bit at a time, but there can be recombination of the genes, because they are segmented, into totally new virus particles that have different properties.
So viruses don't just drift in their genomic sequence. They can have dramatic shifts in their sequences that occur-- whoops-- through combinations of viruses that come-- that have infected different animals. So some of the common strains, when we talk about certain strains that have been very, very troublesome to humans, may result from such a combination.
So this would be the Eurasian pig flu, the classic pig flu, the human flu, the bird flu. If, in certain communities where people often live with their livestock, a cell gets infected with viruses, a human virus and the swine virus, they can mix and match together. And you can make a totally different viral composition, where you've got one piece of genetic information from the swine flu and seven more from the human flu.
And what that can suddenly mean is that the-- first of all, the vaccines don't work at all, but that they may have very, very different properties for infectivity. The protein that is expressed that may cause that very first attack of the virus on your cells in the upper lungs may be very different to the type-- to the protein that comes from the swine flu and may give you much more serious lung infections because they can go deeper into the lungs.
So it can be very small changes by pulling an enzyme, a piece of gene, from a completely different organism and matching it up with the rest of the genes from the human virus that makes for dramatic shifts in viral infections that cause these sorts of sudden tectonic shifts where we've really got to deal with a virus.
There are two terms up here. There's H and N. These are hemagglutinin and neuraminidase. They are two proteins that are in the viral coat, so you'll often hear viruses referred to as H1N1, H3N3, and it's just the variant of those proteins that are in the viruses. See these little terms here-- that's what that means, what type of hemagglutinin, what type of neuraminidase, is on the surface of the virus.
So I am done for today, and next class will be exclusively about the AIDS-HIV virus, where we'll go into that life cycle and also talk about resistance to therapeutic agents and combination therapies.