Flash and JavaScript are required for this feature.
Download the video from Internet Archive.
Description
Beginning with the example of DNA microarrays, Professor Imperiali focuses on some of the applications and uses of fluorescent proteins in biological research.
Instructor: Barbara Imperiali
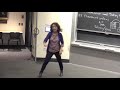
Lecture 28: Visualizing Lif...
PROFESSOR: OK, all right, so just very briefly, I just wanted to remind you because it goes along with the video, bioluminescence is light emitted based on a biochemical reaction. The most common one is the enzyme luciferase, which reacts with a molecule called luciferin to undergo a biochemical transformation that uses ATP. And as a result of that transformation, there is light emitted.
So you don't have to shine light onto these. The light comes from the biochemical reaction. So the assays and a lot of biological work is based on the luciferin, luciferase reaction, so the mushrooms that do this will have both luciferase and luciferin.
So in an organism, you can transfect-- or in cells, you can transfect cells with luciferase, and then when you're ready to do the-- to see the luminescence, the bioluminescence, you can add luciferin exogenously, and there's a number of chemists that are trying to make modified systems that have different light energies and so on.
So there's a lot of protein engineering that's taking place based on the luciferin/luciferase pair. There's all kinds of cool biochemistry going on there, OK?
Anyone who comes in could come and grab a donut. We can move them, or do you want a donut?
AUDIENCE: [INAUDIBLE]
PROFESSOR: OK, all right. I didn't mean to call attention to-- OK, I want to go briefly back to arrays, DNA arrays, because I want to explain to you the power of this technology. So last time, we were talking about arrays which are basically printed layouts, and I'm-- this is going to be a very tiny array with six sites on the array. So it's a two by three array.
The ones that are used in biology are way larger than that. You can see those up here-- far, far more spots, and this is just the size of a glass slide and at each of these sites, there would be different DNA sequences.
So they're specific DNA sequences, and they are printed onto the array with-- it was originally done just with printers. Now there's more technological ways of handling this, and they're usually on silicon or glass slides. So there's a lot of engineering went into the original arrays to make these really packed tight distribution of DNAs.
And these would be what are called addressed arrays. We know what's at each of the sites. We're aware of the sequences at each of the sites, and there's really cool chemistry that would have enabled the disposition of these arrays. So what these arrays are used for is to probe complementary pieces of DNA.
So let's say we've got a GHIJ that come potentially from genomic DNA, and they complement-- each of them complement one of the sites on the J-K-- complement one of the sites on the array, but the DNA that you're screening is specifically labeled with a fluorophore, OK?
So these would be fluorophore labeled, and arrays enable you to do is to rapidly screen either genomic DNA or, actually, much more usefully, as you'll know now, the transcriptome, so the messenger RNAs, and I'm going to remind you about technology to take the messenger RNAs from cells and then make them an appropriate copy of a complementary DNA to what's on the arrays. So let's go take a look at the next slide which describes the experiment.
So let's say you want to screen populations of cells where one set of cells is cancerous and the other one is healthy. You would collect the lysate from those cells. You would collect the messenger RNA presumably based on a tag or a feature of the messenger RNA that's common to just the messenger.
What would that be? What would be common to the mature messenger RNA that would allow you to capture just the messenger RNA, not all the other RNAs, not DNA. Anyone? Yeah?
AUDIENCE: [INAUDIBLE]
PROFESSOR: The poly-A tail. So you can, with an appropriate resin, for example, a poly-T support, you can capture that all, and then in each case, you would take the messenger RNA collection, and then what you need to do is turn it into a complementary DNA sequence.
We're much happier screening at the DNA level because the RNA is less stable. So it's really handy to do that conversion, and you use the viral enzyme, reverse transcriptase. That's an enzyme that comes from the retroviruses that are exemplified by the HIV retrovirus that we'll be talking about in the last week of class. So you'll learn where reverse transcriptase fits in the life cycle of the HIV virus.
Then once you've collected the DNA that's complementary to the RNA from each type of cell, you label them uniquely, for example, with green fluorophores or red fluorophores. It's kind of a global reaction you just paste-- chemically link the fluorophore to each set of DNA.
And then you basically combine everything and take a look at how the chips, how the arrays, light up. So your typical experiment would be everywhere you've got something that has a red fluorophore might bind to a unique site. Everything with a green fluorophore might bind to another unique site on the array.
And then if both types of DNA binding to the same site, that would show up as yellow. If nobody is binding, it would show up as a blank spot. So you get these collections where, pretty easily, you can look at thousands of sequences to see whether they're complementary. That's on your array.
Everywhere you see yellow, the cancer cell and the healthy cell, a binding kind of equally, so there's nothing disease related there. So that's an interesting feature because you can say, well, none of these are problematic segments, but then you see very clearly spots that are clearly red.
So the sequences of the DNA on the glass or silicon chip are complementary to sequences that are unique to the mRNA in the cancer genome, in the cancer cell line from that lyaste. So it's pretty cool.
I don't know if any of you have hit that link, but it's basically this guy is sitting at a bench and doing an experiment where he's able to do thousands-- the equivalent of thousands and thousands of experiments just by using one gene chip to do the probing. In other cases, you will see spots that are green, which mean they come exclusively from the healthy cells.
So those would be really the ones you want to interrogate, and where it's black, nothing's binding, so it's less important. So it allows you to go from a grid of thousands and thousands of different sequences to pick out suspects in a disease-related situation.
Does that makes sense? Pretty cool technology. The original chips were called-- they were from, I think, Affymetrix, the Affy chips, and they are really widely used even to this day, and there are different types of variations, but I think one of the most useful and ones that will remind you of certain things we've talked about in the class is that you can grow cells of different sort of, you know, histories.
For example, you collect the messenger RNA because, remember, the transcriptome is much more interesting to us than the genome. The chips would have to be a lot bigger to scan all of the genome, and then you use reverse transcriptase to make a complementary DNA of the RNA because it's more stable, more tractable to work with, and then you put on a fluorophore label, mix everything together, and see what you get.
So those are my candidate spots. So if you had an experiment, let me think-- walk you through what the arrays can do, but let's make sure we know what they can't do because it's always important whenever someone says, I got this technology. It's going to solve all the problems of the world. Throw away or your beakers and test tubes. You just don't need to do any of that, and you can solve everything with arrays.
So let's say you've got a situation that, in some cases, although genes may be defective, the messenger is still produced, OK? So there's something wrong, but you make the messenger RNA. So it would still kind of show up normally on the chip, but the gene defect prevents translation into proteins.
So you get the gene, but you can't translate it, so it's going to look like a normal gene, but it's in the translation from the track-- from the messenger RNA to the protein where things go wrong. What are you going to see on the array?
Is it going to look healthy? Is it going to look not healthy? What's going to be the outcome? Could I use an array, a DNA array, for this experiment? Yeah? What do you think?
AUDIENCE: [INAUDIBLE]
PROFESSOR: Yeah, Yeah. It's just going to look like everything binds to the chip. It's not going to be informative. So in this case, you need to do very different experiments that are at the protein level, all right? So I want you to remember that these are very good to get the genomic sequence, the messenger RNA sequence.
But if you've got, for example, a protein that is made, and there's a problem with, let's say, a phosphorylation by a kinase that may be critical for function in a cell, you will not see that in the array. That will not be visible. You'd have to do different types of experiments, for example, with phosphoproteins, specific antibodies to tell that there was a disorder in those systems.
So always when someone tells you, I've got this great experiment, you say, what can it do? That is awesome, but what can't it do? OK, I need to worry about that, all right?
OK, so what we've seen so far is we've seen a variety of fluorescent tools, for example, to label the nucleus, either the original ethidium bromide types of stains that will intercalate into DNA and give you a fluorescent signal that could be seen on a gel.
We've seen the evolution of ethidium bromide to less toxic materials, for example, the DAPI stain. So ethidium bromide intercalates-- ethidium-- sorry, DAPI binds in the minor groove. And we saw images of that last time.
One important distinction that is made with these kinds of dyes is that DAPI is what's known as a supravital dye, which means you can use that dye and observe live cells. So supravital is associated with the types of experiments that you can do observing cells that are still alive.
The ethidium bromide is not such a dye, and neither are the antibodies because so ethidium bromide is toxic, so even though the ethidium bromide gets into cells, it actually should have the H here because that would be ethyl bromide in my language.
So ethidium bromide has the H there. You can't use that. It's not a supravital dye because it's toxic to cells because intercalates in the DNA and disrupts replication, so that is not supravital, so DAPI is.
This guy isn't, and antibodies, which we learned a little bit about last time, these are from my description, are simply reagents to recognize components of biological systems, most commonly, proteins, and now we're getting better at making antibodies to carbohydrates or glycans.
Are antibodies supravital or not, and why-- if they're not, why? Could I use that on a cell and follow a live cell with an antibody? Yeah? Carmen?
AUDIENCE: It doesn't seem very likely to me that the antibodies will allow the proteins to do whatever it is that they do.
PROFESSOR: Right.
AUDIENCE: So I think that the cells would die.
PROFESSOR: So it's a correct-- they are not supravital. What do you think would happen if I add an antibody to a living cell? Could it stain-- it could stain something on the cell surface, but it might, as you say, interfere with the function of the cell.
Let's say I have an antibody to the epidermal growth factor receptor. That is going to alter the properties. So you're correct in that respect. What about targets inside cells? Are the antibodies going to get in? Yeah?
AUDIENCE: [INAUDIBLE]
PROFESSOR: Yeah. Yeah. Yeah, it's a big wall, frankly. It's an impenetrable barrier to get in. So you can really only stain in-- you can only observe in what are known as fixed cells. And when we call a cell a fixed cell, we actually mean we've broken it because what we've done is we've treated the cell population with methanol, which pokes holes all over the cellular membranes.
They're fixed. They're static on a slide. They're not going to move around anymore, and you can stain with antibodies. You can tell what happened at the moment-- what was happening at the moment the cell passed away, if you will, what was going on, which proteins were there.
But you can't keep observing moving forward because the cells then are no longer viable, all right? So neither of these approaches are super vital, but they're for different reasons. One is toxicity. The other one is also toxicity, as Carmen pointed out, but it's also a problem with membrane permeability.
So what we're going to talk about in the rest of this lecture is a way to get around this impenetrable problem, and this-- and the discovery of the green fluorescent protein, and we're really mostly about GFP and its close siblings that might be different colors.
What I want to walk you through is the discovery of the protein, and I really want to impress upon you how science happens in funny, small steps early on. You could never have predicted that a jellyfish off the coast of Seattle was going to revolutionize biology, biochemistry, life sciences, right?
Who would have ever thought of that? And so that's why, you know, they colleagues, we and our colleagues, are so excited about fundamental science where you don't quite know where you're going, but you're working on something cool, and then the prepared mind goes, wow! That's interesting. I could use that for this problem.
So Shimamura was a Japanese biochemist, who was fascinated by jellyfish and their bioluminescence and worked for years, slaved away for years and years. Apparently, he would go with his family to the small islands in Puget Sound and have his kids go and collect jellyfish all day long because he noticed certain things about the jellyfish that were rather intriguing with respect to their properties.
And the key thing that was observed was that there is a bioluminescent protein in jellyfish known as aequorin. So that's the kind of luminescence we just described, but in the dark, there was also a fluorescence species, and it turns out that the light energy from a aequorin actually can excite the flourophore in the green fluorescent protein, and then you emit the light of the wavelength in the green.
So it was actually a couple pair of proteins, aequorin and the second thing that was just the green fluorescent protein, and what was fascinating about this protein is more and more work was done is they didn't seem to need any additives.
So for bioluminescence, you got to add ATP, and you've got to add, you know, the luciferin. You've got to add things to see the fluorescence. What was unique about the green fluorescent protein is it didn't need anything to add. You just had the protein, and it was fluorescent.
So what I want to talk to you about is the molecular basis of this fluorescent, and we'll also talk, though, about the protein engineering that was systematically done to make this more and more of a useful reagent. So Shimamura was the first person.
He had his kids collect so many jellyfish that they could extract the green fluorescent protein through traditional old-school biochemical methods and grow crystals, protein crystals. Guess what? They are bright green, and we're able to solve the structure.
Once they had the structure, they sort of knew what was going on with the jellyfish, and they were also able to recognize a particular part of the sequence of the green fluorescent protein that ended up being the precursor to the fluorophore that we see nowadays and we understand.
Originally, the protein was not monomeric. I'll talk to you a bit about that later. For technology, it's much more handy to have this protein as a monomore, not as a dimer or a tetramer. That makes experiments complicated, but I'll show you a very easy trick that was done to fix that.
And so the other people who shared Nobel Prize with Shimamura, who, by the way, passed away just a couple of weeks ago, in fact, but a an amazing old age. It must have been all that digging for jellyfish that helped him live that long.
There was also Martin Chalfie at Columbia, who demonstrated that the gene for the green fluorescent protein could be put in all kinds of other animals, organisms, C elegans bacteria and they would fluoresce. And a really major player in this entire story was Roger Tsien, who died very young of a stroke, who was the chemist-biochemist who put the pieces together said, if this is GFP, we can use it for so many different things. So he really advanced the technologies of the applications.
So protein expression could be-- they realized quite early on could just be programmed into a protein by just having the DNA for the green fluorescent protein stuck on to a favorite protein of interest. Then you would have that DNA be transcribed, translated in the cell, and then your favorite protein in the cell would fluoresce green because it was attached as a construct with the other proteins.
So all of those things became enabled quite quickly. This could be done in all organisms, eukaryotes, prokaryotes. It's pretty non-toxic, so expressing a bunch of GFP in a cell doesn't kill it. That's kind of handy.
So it really is a super vital system, and it's visible in all kinds of tissues. So what I'm going to show you here are some of the seminal results, first of all, the structure we'll talk about and then Chalfie was able to put the DNA into proteins and bacteria, and, also, proteins that were labeled in C elegans.
And the story of Chalfie is kind of funny. He's one of those guys who sort of didn't necessarily have all the equipment he needed, but he knew this was really exciting, and he needed a fluorescence microscope. So he would call up all the companies who sold microscopes and, say, I'm really thinking of buying this fluorescent microscope. This particular one would be-- these are a couple of hundred grand, you know, these are not cheap things.
And he'd talk the company into putting a microscope in his lab for a month as a demo. It's, like, you know, saying to the car dealer you want to buy a car and then driving a Ferrari around for a month and then saying, it's not really going to work for me. So he collected all his early data on microscopes that were on loan from Olympus and Leica and various other places. So that's a funny, funny twist.
OK, so the fluorophore-- all right, so they could go from DNA to protein sequence. Then they could look at the structure and see that the place in the protein sequence that went serine, tyrosine, glycine. So here we go-- serine, tyrosine. You recognize that one. It's the one with one of the aromatic rings, glycine is the one with no substituent.
It was common in a number of organisms, mostly aquatic organisms that fluoresce and seem to be the origin of the flourophore in GFP, and so the chemists got to work, and you know what chemists do, they start drawing arrows and joining bonds and figuring out how can we go from something that's basically dead, and it's not got any fluorescence at all for something that's fluorescent.
And so from the structure and from working out the mechanism, they basically found that this little piece, this tripeptide within the entire structure of the DNA primary sequence, was able to cyclize through that nitrogen attacking that carbon and then an elimination, and then there was an oxygen-dependent oxidation to give you this structure.
So if you look at this, you can still see the serine. You kind of know this was tyrosine, but it doesn't look like it anymore, and it turns out the glycine was incredibly important. It doesn't look like it's involved in the chromophore, but when you have a glycine in a sequence, it allows you to do some funny twists and turns because it has no substituents.
So it allowed that loop to form. Once that loop is formed, chemistry can occur. If the glycine wasn't there, things might be not in an ideal situation to form the fluorophore. The oxidation to put in that double bond is oxygen dependent.
So in some cases, the fluorophore would mature a bit more slowly. So you would go from the free state that's not fluorescent to the fluorescent state quite slowly if you withheld oxygen.
So a lot of engineering was done to improve the maturation time, to improve the photo physics. There's really cool photophysical experiments that were done, but that's the basis of it, and the emission of this flouorophore, you can almost recognize that it's a fluorophore because it's got a lot of what's called conjugation in organic chemistry-- one double bond, another double bond stuck onto this ring with multiple double bonds.
And it emits at the same-- a similar wavelength to the fluorophore fluoroscein, so people were happy because all the filters on their microscopes were the right ones. If you just used the fluoroscein filters, you would get a GFPC. So that really was made in heaven for those guys.
OK, so let's take a look at this. Here's the wire diagram of the original crystallized structure. I've planted a ribbon on there. I get rid of all the side chains, so you see this beautiful dimer structure.
Let's throw away one of the dimers, and you can start to look. There's a little something sneaky in there, and as you get closer, you can start to see the structure.
There's this sort of thread going through it. That's just a trace of the backbone, but there is the structure of the fluorophore. Here's the fluorophore with the space filling, and what I think is kind of cool is if you kind of twist the GFP around, it's kind of like a barrel, and it looks like a bird in a cage because it's caged in there.
And to be honest, it's the structure. It's not just the structure of the flourophore. It's the fact that the fluorophore is inside a kind of hydrophobic environment that makes it fluoresce. I could go into the lab and make that flourophore and have it in a beaker of methanol, and you wouldn't see anything.
It's only in that environment that's created in the GFP molecule, so it's fascinating. The structure of GFP creates the shape to cyclize, and it creates the environment for good fluorescence. So he has a mass of animals that have been labeled with GFP. As you've seen, our mouse has been labeled multiple times.
This was the first GFP rabbit. He was known as Alba. He even had his own name, but there's seaweeds and zebrafish and mice from a letter, C elegans, the famous, the fly. What else? I don't even know what these things are, but Purkinje cells and so on.
Anyway, so this shows you the universality of the tool. A brief mention about the dimer problem, people looked at the structure of the diamond from the crystal structure and found that there was a sticky face between two monomers of GFP that encouraged this quaternary structure.
And so they simply changed two alanines that were sitting right at the dimer interface, one from one monomer and one from the other, created a sticky patch. Sounds a bit like hemoglobin, if you remember, the sickle cell hemoglobin.
They changed them to lycines and [INAUDIBLE] solved you had just monomeric protein because when you do a lot of experiments with GFP, you don't want GFP randomly dimerizing because it's going to change the biology of the protein it's attached to. So this very, very easy structure-driven engineering was perfect.
OK, so let's take a look at some of the early things that could be done now. We take it all for granted, but GFP can be used as a reporter gene to do a number of things in cellular systems. So, for example, if you want to study a regulatory sequence to know whether this promoter works well, you can put it at the front end of the GFP gene and then see if the promoter works under certain conditions, then the GFP will be produced. The cells will grow green.
And this is a nice variant to a luciferase-based system or a LacZ based system where you're actually having to treat and fix things in order to do the LacZ experiments. So here's a really nice example with C elegans.
What you could do pre-GF-- we really have-- it's like ADBC-- you know, anti whatever before year 0 and after year 0. The biological world is pre GFP and post GFP, frankly, the impact that it's had on the system.
So here is a C elegans. As you know, all the neurons have names, and a lot of C elegans biologists are very familiar with each, you know, single cell within the C elegans, in particular, the neurons. So this is a listing of the touch receptors.
You could have an antibody to those, and so you could do some antibody staining, but antibody staining, remember, we got to fix the worms. They don't like that. You literally fix them down and do the staining with the antibody to get inside the C elegans to see the antibody light up the proteins.
You could do beta-gal activity, same thing, but in both cases, these are dead C elegans. They're fixed. They're in place. You can't observe them going forward. But look instead, that the images you would get with GFP, where you could totally pick out all the touch receptors.
And this is a situation where an important protein in those cells MEC-17 is co expressed with GFP, and you can see this beautiful image, and the worms are still alive. You could totally watch what was going on with them, and he just wants you to know that he's alive and well and kicking.
OK, now, progress has to happen. So one green protein does not a history make. So, immediately, with the identity of the structure of GFP, I am going to go back really quickly to look at that picture of the structure for you. Sorry about this back and forward.
OK, the chemists realize that if they change this tyrosine to some of the other amino acids that looked a little bit like it, like phenylalanine, tryptophan, and histidine, they might alter the photophysics of this structure because if you put tryptophan there, there's even more double bonds. If you put phenylalanine there, that OH is gone.
So there's really opportunities, and that's the chemists saying, I can look at this protein structure, and I know the one thing I can change is to change that amino acid back at the DNA level. So I'm going to change the codon for tyrosine to the codons for tryptophan, phenylalanine, and histidine and then see what my fluorescent protein looks like, OK? Does that make sense?
So it was the one thing that's obvious. I can fix this. So what they did was they made the series of GFPs, and they transfected cells, and they were able to see that when you replaced various residues, for example, tyrosine to tryptophan, tyrosine to histidine.
There's a little bit of variation here, and don't worry about this for a minute. They were able to create bacteria that had been transfected with the mutated GFPs in order to give them different colors of bacteria.
So my question to you is, if you look at these, this would have been the original GFP, except made a little bit brighter by a small substitution, which of these would emit at the shortest wavelengths. So just look at the colors, which is the shortest wavelength, which is the bacterium that expresses the protein that emits at the shortest wavelength.
You'd always be provided a picture of the electromagnetic spectrum. Yeah, out there.
AUDIENCE: The GFP.
PROFESSOR: Yeah, the blue one, exactly. So you can look at the spectrum, and you say, OK, I've got blue down here. There's that cyan. It's kind of a blue green, and then I move towards the more yellow.
So you can pick it out and say, it's the lowest energy. It's the shortest wavelength emission, the highest energy emission. OK, all right, and these were the variance that I just described while I went back to the picture.
So you could make a blue one with histidine. You could make a cyan one with tryptophan. You could preserve the green one with tyrosine, but a change in the serine to a threonine just to improve the photophysics, a long story. I won't-- I'm happy to chat about it.
And, in fact, there was one clever one where you couldn't really make the protein yellow, but if you had the tyrosine nearby sandwiched with the tyrosine and the GFP chromophore, you could actually go as far as the yellow flouorphore.
And that, in principle, should make everybody really thrilled. It's always this situation with protein engineering where you make something, and it's a huge improvement and then everyone says, well, what else, you know? What's next? We need red dyes. We need all kinds of different dyes.
So teams went back to the oceans and actually collected organisms based on the color they fluoresced at, nice way to sample things. And, actually, we were able to ultimately discover a red fluorescent protein from the Discosoma coral, the original protein, DS red, and if you look at this kind of carefully, it looks a lot like GFP.
There's the tyrosine, that funny ring. There's a double bond there, but there is an additional double bond down further into the sequence that extends what we call the chromophore. And when you extend the chromophore, you're more likely to move to longer wavelengths, and that's how they got the red, and then a bunch of engineering later, they got what's called the fruits, which emit at all different wavelengths.
Much of this was not done rationally because they went to nature. They found out what nature did. They got here, and then they did a process known as gene shuffling, where they mixed and matched portions of genes to get them the entire color spectrum.
They're not all fabulous fluorophores. They have some problems. They bleach easily and so on, but, nevertheless, this is a truly amazing sort of outcome. OK, so and they enable you to do art work with fluorescent proteins with bacteria, so you just paint your picture and wait for the bacteria to grow, and you would have all those colors.
So fluorescent proteins originally were very limited, originally just green, then blue-green and greenish yellow, but, now, with the palette that's available, you can color all kinds of organelles in different types of colors, all right?
OK, so now let's take a couple of things. When we opened up this class, we showed you a bunch of these pictures because we wanted you to stay in 7016 with us to see the cool things you'd see, and they were pretty much what we would call eye candy.
They look cool, but how did we make them? And, now, with the knowledge of GFP and RFP and so on, you can say, OK, I understand what this experiment is, and you can certainly see that this is a super vital technique.
So what I want to ask is, what are we looking at? What is labeled to give us this beautiful picture? So you can sort of go down, and there's a bunch of options, but, obviously, anything where you think you're labeling DNA with a fluorescent protein is incorrect. We're labeling at the protein level.
We're making the DNA to express the fluorescent protein, but we're actually labeling a protein as opposed to DNA. So A and B are out for sure, but as we go through them, you can start to see the chromatin is red and the tubulin is green, so the outcome there would be, whoops, what we're observing-- I thought what we're observing is that the histones, which are the proteins associated with chromatin, are labeled with a red protein.
And you can see that there. Those are the chromatids, and the tubulin, these green fibrils, is associated with a GFP. So C would be the correct answer there.
And there's another one of these cell division pictures. Once again, the same sort of idea, but what I think is really cool here, as you can literally see, I love this picture because there's this poor chromosome. It doesn't seem to be able to get with the team.
He's hanging down there, and, eventually, just before cell division, it seems like things work out OK and cell division happens.
Now when you think of this, the capacity to watch this stuff in action is really pretty amazing. This was another one. This a set of cell lines where proteins in cell cycle are labeled.
You had a question on exam three about proteins building up and going-- and going away during parts of the cell cycle. So, oftentimes, the degradation of a protein occurs when a protein gets labeled with ubiquitin by a ubiquitin ligase, and then it gets destined for degradation.
So the proteins that you're seeing grow up in phases of the cell cycle are going away because of the ubiquitin proteasome system. So in these cells, particular proteins have been labeled, and here you can see the phases-- the components of the cell cycle as proteins come and go through cell division, and you can literally observe where in the cell cycle each individual protein is.
Why is this so fascinating, I think, in therapeutic development is that you could literally have drugs that might impact aspects of the cell cycle, and you could watch them in real time impact the imaging that you see on this screen with the red and the green because if you arrest at a certain stage in cell cycle, the cell will get stuck at a particular color, and you would even know which phases, which parts of the cell cycle, are being impacted.
So I think that's a really sort of captivating way to think of what you can do with these proteins because you can see things in real time, dynamics of cellular systems. And I think, oh, and I'm going to finish a few minutes early, but I looked desperately for a fluorescent turkey, and I couldn't find one.
So I went with this light string turkey and fluorescent colors. It seemed to fit the bill. All right, so please help us finish up the bagels. Make sure you guys over there get them and have a good break, and I'm passing you back to Professor Martin for Monday, which will be a continuation of imaging, OK.