Flash and JavaScript are required for this feature.
Download the video from Internet Archive.
Description
After completing the topic of protein trafficking, Professor Imperiali introduces cell signaling. In the first of two lectures on this topic, she covers the paradigms and mechanics of cell signaling.
Instructor: Barbara Imperiali
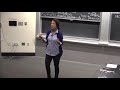
Lecture 20: Cell Signaling ...
BARBARA IMPERIALI: Now, I want to talk today about one small thing before we move on to signaling, because it really kind of completes the work that we talked about with respect to trafficking. So I popped this question up last time, and it seemed like there weren't quite enough sort of people leaping to give me an answer. But let's just take a look at the big picture of things, as it's always good to do. Because this will also get me to one other topic, which is protein misfolding.
So at the end of the day, what really defines where a protein is, what it does, is defined by its sequence. But you always want to remember that a protein sequence is defined by its messenger. The messenger is defined by the pre-messenger. Yes, there may be splicing events that really cause changes in localization. But the pre-messenger includes the content. And then what defines that is the DNA.
There's certain aspects of regulation at the epigenetic level that we don't talk about barely in this course. But I want you to make sure that you realize at the end of the day, what the protein is, how it folds, is defined originally by the sequence of the DNA, although a long way along here. The post-translational modifications that we started talking about last time are defined by the protein sequence, which all the way is defined by the DNA-- so, so much of protein function.
And there's one more aspect of proteins that's defined by the DNA sequence, and that's whether a protein folds well, or perhaps, in some cases, misfolds. And that's the thing I want to talk about very briefly today. Because I think that this captures the picture.
So let's just go over here and write misfolded proteins, which, just like everything else, largely end up being dictated by the DNA. Because whether a protein folds faithfully into a good structure or misfolds can be a function of the protein sequence.
So there could be mutations in the protein that ultimately end up that the protein misfolds and forms either a misfolded tertiary structure, or even worse, adopts an aggregated form that causes a lot of damage within cells and outsides of cells. So I want to talk just briefly about the processes that we have-- it's just one slide-- to deal with misfolded proteins.
So when a protein is translated, it almost starts folding straight away, especially large proteins. A fair amount of a protein may have already emerged from the ribosome and started folding, even when the whole protein isn't made. The sequence ultimately ends you up with a well-folded protein.
But if the protein does not fold fast enough, or there is a mistake in this, which might be caused intrinsically by the primary sequence, if there's a mistake in that-- so slow folding or incorrect folding-- then you will end up with a protein that's partially folded within the context of a cell.
We especially encounter misfolded proteins when we are overexpressing proteins in cells, because you're just making one of a type of protein really quickly, and it doesn't have a chance to adopt its faithful structure.
So there are proteins within the cell that helped sort of protect the folding process early on, to allow the protein to have enough time in singular, not with a lot of copies of itself around that are misfolded, to adopt a folded structure. And these proteins are called chaperones.
I don't know if you guys are familiar with the term chaperone. It was a term that was heavily used in the sort of 18th and 19th century. A chaperone used to be an aunt or someone who you would send out with your beautiful young daughter to chaperone her, to protect her so she didn't get bothered by those mean men out there. So chaperones were-- that was the original definition of the chaperone. And it's kind of interesting that the chaperones are now proteins that help folding or protect against misfolding.
How do they do this? Generally, a protein will fold poorly if it's very, very-- if it's quite hydrophobic. And hydrophobic patches are exposed in aggregate.
So let's say, you have a protein, and there's a lot of copies, but they're not folded. If you have things that are hydrophobic that would normally end up tucked inside the protein, if the protein hasn't folded in its good time, these will just start to form aggregates, sort of associating with each other. It's just a physical phenomenon.
If you put something that's got a lot of hydrophobic faces on the outside, this will start forming an aggregated bundle, and not a nicely folded protein at all. What the chaperones may do is in part hold the partially folded protein. So let's just think of this big jelly bean as a chaperone until things start to adopt a favorable state.
But sometimes it's just too much. The chaperone cannot handle the flux of protein. So the protein ends up being recognized as misfolded. And then it gets tagged as a misfolded protein, and it gets taken to a place in the cell for disposal. So if you are unable to fold, there is a tagging process. And I mentioned it last time. It's a process known as ubiquitination.
This is also a post-translational modification, but it's one that occurs on poorly folded proteins. And I'm going to describe to you that system, because the ubiquitination is the flag, the signal, or the tag, to take this protein to the great shredder, basically. And so what does the-- what's a paper shredder-- I like the analogy with a paper shredder.
So here's a fellow who's got too much on in his inbox. So he just sends it straight to the shredder. It's a little bit about too much misfolded protein being made. So instead of sort of waiting to deal with the paperwork, you just send it straight to the shredder. And the proteasome is the cellular shredder that actually breaks proteins up into small chunks, and then digests them out.
So think of the proteasome as a shredder, which chops up proteins into small pieces, mostly into short peptides that are 8 to 14 amino acids in length-- fairly small. Short peptides won't cause a problem in aggregation, and will then be further digested.
Now, if you've got this shredder sitting around in the cell, it's like having a paper shredder on all the time. You've got a-- there's a risk things may end up in there without meaning to be. So for things to be tagged for shredding, they go through what's known as the ubiquitin system.
So the first thing to get into that is-- and it's only then that proteins are tagged for shredding up or chopping up by the proteasome. So as you can sort of tell by its name, it's got protease function, but it's a large, macromolecular protease, with lots and lots of subunits that are important to cut that polypeptide into smaller pieces.
But because many of your proteins may be partially folded or misfolded, they first have to be unfolded. So the ubiquitin is the signal to send proteins to the proteasome, where the second action is protease activity, and the first action is unfolding.
So what I show you on this picture is the barrel structure of a proteasome. Let me explain the components of it. The red component of the proteasome is a multimeric ring that uses ATP and starts tugging apart the protein that you need to destroy. But it will only do that if the protein becomes labeled for destruction by the ubiquitin system.
And I am showing you here a massively simplified version. Let's say this is a misfolded protein. It gets tagged with another protein. It's a really little protein known as ubiquitin. I've shown you the three-dimensional structure here. And using ATP, you end up managing to put a ubiquitin chain on the protein that's going to be destroyed. That is a post-translational modification that is a tagging for destruction.
If the protein is not tagged, then it's not going to be chewed up. That makes sense. You don't want to be chopping up proteins in a cell with wild abandon. Once the ubiquitin chain is on here, the protein will bind to the unfoldase part of the proteasome, and with ATP, it will just stop tugging the rest of the residual structure apart to thread the protein down into the blue part of the barrel.
It's a little hard to see it like this, but it's literally, the proteasome, are four concentric rings. Let me see. I hope my artwork is going to be good enough. Well, that's an unfoldase. And so is that. And then in the center, there is a protease. And each of these components is multimeric, having six or seven subunits.
So it's a huge structure. It has a sedimentation coefficient of 20S, that entire structure. I don't know if you remember when I talked about ribosomes, they were so big we didn't tend to talk about them by size. We talked about them by sedimentation coefficient. And the large and small subunits of the ribosome, the eukaryotic one, just to remind you, were 40S and 60S.
So just remember that S stands for Svedberg. It's a sedimentation coefficient unit. It describes how fast approaching precipitates. So once the protein has been labeled with ubiquitin, it binds to the unfoldase. And then the single strand feeds into the center core, which is two sections of protease. So it's feeding in here. It sees the protease activity. And then it's just short pieces of protein are spit out of the proteasome.
Once these are really little pieces of peptide, they're readily digested by proteases within the cell. And you can recycle the amino acids, or you can do other things with these small pieces of peptide. They actually end up sometimes being sent for presentation on the surface of the cell by the immune system. And you may hear a little bit more about that later.
So the proteasome-- oh, I apologize-- this should have been 26S-- has a molecular weight that's very large-- 2,000 kilodaltons. That's why we refer to it by its sedimentation coefficient. So this machinery is very important to get rid of misfolded or aggregated proteins to destroy them.
Now, does-- are people aware of the sorts of diseases that can result from misfolded proteins? Has anyone been reading the news much about certain types of diseases, particularly in neurobiology? Anyone aware of those? Yeah.
AUDIENCE: Was it mad cow disease?
BARBARA IMPERIALI: Which one?
AUDIENCE: Mad cow.
BARBARA IMPERIALI: Yes. Mad cow. So there are a variety of neurological disorders, and mad cow is one of them. Creutz U-T-Z feldt-Jakob. But Alzheimer's disease is another one. Pick's disease is another.
There are a wide variety of neurological disorders that result from misfolded proteins, both inside the cell and in the extracellular matrix, forming these tangles that are toxic to the neurons, causing them to no longer function, and then resulting in many of these neurological disorders.
The ones I've described to you, I've mentioned to you here. I know many of you are familiar with Alzheimer's disease. Mad cow disease is a variant of a particular protein misfolding disease that was first noted in cattle. And they basically just fell down, dropped down. And it was in some cases ascribed to-- the contagion with the disease is ascribed not to a virus or to a microorganism, but literally, to misfolded proteins causing the formation of more misfolded proteins.
So these are all collectively designated as prion diseases. I think you'll have read that term. And it's a particular kind of disease that the infectious agent isn't a living system-- not a virus, not a microbe, a fungus, a protozoan, but rather a protein, where it's misfolded structure nucleates the formation of more misfolded structure that leads to the disease.
So I grew up in England during the years where there was a lot of mad cow disease in England. And even though I'm a vegetarian in the US for 30 years, I can't give blood in the US, because I lived in England during the time when there was a lot of mad cow disease. And this can be dormant for a long, long time before it suddenly takes over.
So there's restrictions on blood donation in certain cases. And it's because it's not something you can treat with an antibiotic, you can treat with an antiviral. It's literally traces of badly folded protein that can nucleate the formation of more badly folded protein, that can lead to the diseases.
These were-- there's particular instances of some of these diseases in tribes where there's pretty serious cannibalism, and eating your sort of senior relative's brains was considered to be something-- an important act of respect. And there was this transfer of some of these prion-type diseases through cannibalism as well.
So eating contaminated meat, be it a cow, be it your grandparents, whatever, it's something that actually is-- it's a serious transmissible disease. And it's really-- in the situations where it can be sort of related back to contaminated meat are one thing. But there are variations in the case of Alzheimer's, where the sequence of proteins may dictate that they don't fold well, or they're not post-translationally modified properly, so they end up as misfolded proteins.
So these are often genetically linked disorders, some of the things like Alzheimer's. And once again, remember that goes all the way back to the DNA, which might, in some cases, trigger the misfolded disease.
So it's a fascinating area, and there's a tremendous amount to be studied. Because of the aging population, these diseases are piling up, and we need to mitigate the causes of the disease, and find ways, for example, to slow down. If there are these fibrils of protein that are misfolded, can we maybe inhibit that formation with some kind of small molecule inhibitor to mitigate the symptoms of the disease? So it's a very, very active area, because almost every-- many, many neurological disorders seem to be coming down to misfolded proteins.
So let's move on now to signaling. All right. So we're going to spend two lectures on-- the remainder of this lecture plus the next lecture. And what I want to do in this lecture is introduce you to some of the paradigms, the nuts and bolts, the mechanics of protein signaling. And then in the next lecture, I'm going to show you examples of how all the characteristics that we define signaling by get represented in signaling pathways within cells.
So I'm going to give you all the moving parts, and then we'll move forward to see how the moving parts might function in a physiological action, such as a response to something particularly scary, or as a trigger to do-- for the cells to do something different.
So let me take you, first of all, to a cartoon-like image of a cell. And we're going to just take from the very simplest beginning. But then this topic will get quite complex, as you see. But that's why I think it's important to reduce the process of protein signaling down to simple aspects of it that we can really recognize, even in much more complicated pathways.
So in protein cellular signaling, this is a complex system of communication that governs all basic activities of the cell. There are no cells that don't do signaling. Bacteria and eukaryotic cells may do signaling slightly differently. But they still do have an integrated correlated system that's responsible for triggering functions of the cell through a series of discrete steps.
So protein signaling can be dissected into three basic steps, where you, first of all, receive a signal. And we're going to talk about what that signal is. What's the nature of that signal, is it small molecule, large molecule? Where is the signal? Where does it act?
Then the next step is to transduce the signal. And finally, you have an outcome, which is a response. So we're going to talk about each of these components in order to understand flux through cellular signaling pathways, and how they work to give you a rapid response to a necessary signal.
All right. So in this cartoon, let's just, for example, think about what if we want to trigger cell division? We might have a signal, which is the yellow molecule-- a small molecule, large molecule. We'll get to that later.
There's a cell here, where on the surface of the cell is a receptor. And that would be the entity that receives the signal. So in the first step in the process, there's a buildup of a concentration of a signal. And it occupies the receptors on the surface of the cell, and in some cases, inside the cell. We'll talk about a bifurcation there. But really, a lot of cellular signaling is dominated by cells coming-- by signals coming from outside the cell.
What happens upon this binding event is the transduction. If you bind to something on the outside of the cell, as a consequence, you might have a change on that structure. If it crosses the membrane, you might have a change on that same molecule structure that's on the inside of the cell.
So that's why it's called transduction. You're transducing a soluble signal from outside, binding that signal to the cell surface receptor. And the cell surface receptor is responding in some way.
And there are two principle ways in which we respond to extracellular signals, and we'll cover them both. The next event that might happen is through the change that happens to the intracellular component of the receptor. There might be a change, a binding event, another step occur within the cell. And as a function of that, you get a response. All right?
So it's really-- thinking it in these three components is a good way to kind of dissect out the beginnings of the complication. And then what we'll be able to do is really start to see what kinds of molecules come in? How are they received? How is the signal transduced? And what's the ultimate outcome with respect to a response? Everyone OK with that? All right.
Now this is what you have to look forward to. So we give you something with three moving parts, and suddenly we show you something with sort of, you know, 100 moving parts. And cell biologists very, very frequently look at these maps of cells, where what they're looking at with each of these sort of little acronyms or names, all of these are proteins, where they have been mapped out through cell biology and cellular biochemistry to be existing in certain components of the cell.
And what has also been mapped out very frequently is, who talks to who? So the fact that JAK S might interact with STAT 35 and so on. So much of this was worked out through cell biology and biochemistry, and also by genetics.
So Professor Martin has talked to you about identifying a player in a complex system by genetics. Let's say you have a cell that fails to divide. You might perhaps screen or divides unevenly, or has some defect in cell division. You might be able to pick out a particular player.
Now, the key thing I want to point out to you with this cell is what's on the outside of the cell and runs across the membrane, and might have the chance, the opportunity, to have both an extracellular receptor and an intracellular function. And those key proteins are things like receptor tyrosine kinases. And we're going to talk about all of these in a moment.
G protein-coupled receptors, and various other cell surface receptors-- so all of these-- anything that spans a membrane has the opportunity to be an important component of a signaling pathway. Because what you're routinely trying to do is have your signal recognized on the outside of the cell by something that spans the membrane. The signal will bind to that. And then you will have an intracellular response.
So that's breaking it down. That's why proteins that are made through the secretory pathway that we talked about in the last lecture, that go through that endomembrane system, and end up being parked in the plasma membrane are so important.
Other proteins that actually get secreted through that pathway are also important. What do you think they may be important for? Let's say you've made a protein within the cell. It goes through all the system. It doesn't stay parked in the cell membrane. It actually gets released from the cell. What might that be doing?
AUDIENCE: [INAUDIBLE]
BARBARA IMPERIALI: Yeah. Exactly. So that endomembrane system that I described to you, that pathway is great for making receivers. And it's great for making signals. And that's really what can sort of fuel the functions of cells. OK.
So in systems biology, you may have heard this term quite frequently. Systems biology is research that helps us understand the underlying structure of signaling networks. So a lot of people who have common interests in engineering, computational analysis and cell biology, might bring in data to allow them to make models of cellular systems, to understand flux through signaling pathways.
So they may make fundamental measurements about the concentrations of some components within the cell. And then try to say, OK, I know based on everything I've measured that this is a dominant pathway for gene regulation. And I could control this pathway by different-- by sort of different types of interactions.
In this cellular system, I also show you another component, which is the nucleus. And when we discuss and describe specific cell signaling networks, in some cases the signaling network may involve receiving a signal, undergoing a variety of changes in the cytoplasm, but then a change that eventually results in a protein going to the nucleus. And oftentimes, those proteins that run into the nucleus are transcription factors that then trigger DNA replication or transcription. And then promote activities.
So this is how you think about it. When you think of cellular signaling, it's really about, what does the signal need to do? And what's the pathway that I follow to get there? So all of those are membrane proteins.
So now let's look at the canonical aspects of signal transduction. So the first-- and I'm going to rely on these little cartoons. But I want, both in this lecture and the next, to really show you where these recur in so many systems. So to that purpose, I want to talk about the characteristics.
So the first critical characteristic is a signal and it's specificity. So a signal will be something that comes from outside of the cell. It could be a hormone that's produced in the hypothalamus and sent to another organ. But the most important thing about the signal is that that signal, which binds to a receptor in a cell membrane, is specific for a particular receptor, and the different signal won't blind to the same receptor. You have to have faithful signal specificity to trigger the right function.
So if it's a hormone, it's got to be the hormone that you want to trigger the receptor, not a related but different-looking structure. If it's a small protein, you want it to be the exact one that binds with high specificity to a receptor.
So what that means is if something is binding-- if a small molecule is binding to a protein on the surface of a cell with high specificity and high affinity, it means that even at a low concentration, it will make that binding contact. But all the other small molecules that are around won't crosstalk into that triggering that interaction. So we have high specificity, and we gain that specificity through macromolecular interactions, just like the ones we talked about in biochemistry.
So if we have a small molecule or a protein bind to the receptor, it's making all those hydrogen bonding, electrostatic, noncovalent types of interactions with high specificity, so that a low concentration of the signal molecule is efficient for binding to the receptor to trigger the function.
The next characteristic is amplification. Now let's put some lines between these guys. Now, with all the signaling pathways that you're going to see, we're going to be looking where in a pathway you get amplification. Very commonly, you might have a response that's just the result of a single molecule binding a single receptor.
But at the end of the day, you might want a large response. You might want to make a lot of ATP. Or you might want to replicate all of the genome. So you need some kind of amplification where in a sense you're turning up the volume on your signal. And you need to do that rapidly.
So frequently in signaling pathways, you go through a cascade of reactions where the signal might affect an enzyme. But once you make that enzyme active, it might work on many, many copies of another enzyme. And then each of those may work on even more copies.
So that's what I mean by amplification, where at some stage you've generated a molecule that can result in the cascade of a reaction. So we often refer to these as cascades. So if you're Spanish-speaking, cascada. You want to think about a waterfall coming from just a single molecule of water. You're getting a large increase in your signal as a result of amplification.
The next feature or characteristic of signaling is feedback. At the end of the day, if you're signaling, I got to make some ATP. I got to run out of the woods. I'm getting chased. At a certain stage, you need to stop all of the process occurring. So feedback is just a negative feedback loop that might slow down some of those steps that are involved in amplification.
So for a pathway, you only want the pathway turned on for a prescribed amount of time. And then you want to be able to say, I'm done with that whole pathway. I don't need to keep churning through all those enzymes. It's time to stop that. And that usually occurs through negative feedback.
And remember, we talked about negative feedback when we were talking about enzyme catalyzed pathways. So feedback is very often some kind of negative feedback, which suppresses a series of transformations, perhaps through a product of those transformations acting as an inhibitor on an early step.
And then finally, the other component of a signaling network-- if you think of signaling networks as electronic structures, you have integration. So that's the last characteristic feature. And let me go back to that big circuit diagram quickly to show you an example of integration.
So if you look at this signaling pathway, all these signaling steps are not single. You just have a signal come in, and you end up, for example, in the nucleus. But rather, other components may have crosstalk within one pathway, and start out either amplifying or turning down a particular signaling pathway. So these are networks. They're not pathways. They're networks that interact and communicate, all to amplify signals or turn down signals.
So integration is an important part of signaling, because you're often dealing with the integrated function of a number of pathways to get a particular response. And that actually ends up being one of the situations where sometimes a particular enzyme may look like a perfect target for a therapeutic agent.
But if you don't take into account the integration steps, you may be trying to deal with a-- you may think you're dealing with a single pathway, but you're, rather, dealing with crosstalk with a lot of other pathways. And what often happens in a cell is there's compensation from other pathways. Is everybody following? Any questions here about this?
So what I want you to think about is that it's just amazing what is orchestrated to have even the simplest functions in the cell, how many interacting components there may be.
Specificity, amplification, feedback, and integration-- all right, so let's talk briefly about types of signals and how we name them, where they come from, in order to make sure we're all on the same page with respect to the language that's used.
So now, signals may take different molecular forms. They may be small molecules, for example, an amino acid or a phospholipid-- just something little. Alternatively, they may be proteins. They may be carbohydrates. They might take different forms in terms of their molecular structure. But we tend to describe signals by where they come from.
So what I've shown you here is a picture from the book that just describes how we refer to certain signals. So there are four different terms-- autocrine, juxtacrine-- and I'm going to just give you a little hint to how to remember these terms-- paracrine, endocrine. OK. So these don't tell you anything about the molecule. They tell you about where it's come from.
So an autocrine signal is a signal that may come from a cell, but it's signaling to itself. So it may produce a component that's released. And so it's producing this through a secretory pathway. It's a release, and it stays in the vicinity of the cell. So the self is self-signaling. So whenever you see something auto, you just want to say, oh, that means it's coming from the same cell where the signal occurs.
Let's move to the next one, which is paracrine. I'm going to talk about jux-- and that's usually from a nearby cell, not a cell that's in contact-- definitely a different cell. So paracrine is-- we would always call nearby. And endocrine is completely from somewhere else, so perhaps coming through the circulatory system. One cell may release an endocrine signal. It may weave its way through the vascular system, and then target a cell. So endocrine is always from a distance.
And juxtacrine is the only one that's a little odd. It's really from cells that actually are in contact with each other. So it's not self-signaling within a cell. It's not a cell that's nearby but pretty close. It's actually physically making a contact. And so that's the last terminology there.
So hopefully, I can get this calcium wave to show you. This is just a video of juxtacrine to signaling. I just want you to sort of keep an eye on things. It's usually a cell. What you're observing here is a dye that lights up in the presence of calcium flux. It's called Fura-2.
And so when you stare at these for long enough, what you can notice is that when one signal will often come from an adjacent cell right near it-- so there are long prostheses. You're not looking at the entire cell, but they're definitely-- for example, this little duo down here, they keep signaling to each other. And that's just a juxtacrine signaling, because the cells are in the contact.
So that just shows you the difference there. If it was autocrine, you just have a single cell responding. If it's paracrine, they would be at more of a distance to each other. I hope that imagery-- this is from a website in the Smith Lab at Stanford.
OK. And then the last thing I want to give you an example, there are many, many hormones in the body that undergo endocrine signaling, and so one example I thought I would tell you about, you all know that insulin is made in the pancreas. It's an important hormone for regulating glucose levels. And it's actually-- functions at the muscle level. So insulin is an example of an endocrine signal, because it travels a distance from where it's made in the body to where it functions in the body. All right.
Now-- so we've talked about the types of signals. Let's now move to the types of receptors. Now, we cover both the intracellular and the cell surface receptors. But we really will focus a lot on the cell surface receptors.
I just want to give you a clue that not all signaling is cell surface. So what I've shown you here is a cartoon where you see signaling, where a signal comes from outside the cell. It goes into the cell and triggers a change. And then the majority of the time we'll talk about these receptors that are in the plasma membrane. And they have an outside place where the signal binds, and they trigger a response inside.
And it's only very specific signals that are able to signal intracellularly, that is, to cross the membrane to get inside the cytoplasm to do the triggering. What kinds of molecules can cross the membrane easily? We talked about that before, when we talked about getting across that barrier. Yeah? Nonpolar. OK.
So you can look at a-- you can stare at a molecule, and if it's very polar or pretty large, it's not going to be able to sneak through a membrane. So something like a steroid molecule, a large, greasy molecule, can definitely make that transition. And so those are the only types of signals that we can really do inside the cell, because they can get across the cell. Many, many other signals have to go through this-- bind to the outside of a cell, and transduce a signal to the inside of the cell.
So one very typical signal that can bind to an intracellular receptor is a steroid. So remember when I talked to you about these lipidic molecules, things like testosterone and cortisol. These are very hydrophobic molecules. So they literally can cross from the outside of the cell without a transporter. So for example, the hormone cortisol.
And when that functions, it just-- an amount of it becomes available, for example, in the bloodstream. It crosses the cell, and it binds to an intracellular receptor. Once it binds to that intracellular receptor, this disengages a different kind of chaperone protein that's keeping it stable. Once it's found, it can then go into the nucleus and trigger transcription. So this is the one example of an intracellular receptor that we'll talk about.
I just wanted to show you a little bit about the steroid receptors. These are molecules that are very-- these are macromolecules, proteins that are very-- quite a complex structure. But they can literally-- and I'll show you the picture at the beginning of the talk next time-- they can literally engulf these proteins. So once the steroid is bound to that, it completely changes shape. And that's what enables the change for it to be triggered and sent to the nucleus.
Now, the key types of receptors that we'll focus on, though, are the cell surface receptors. And there are three basic classes of molecules that occur in the plasma membrane that are critical for cellular signaling. They are the G protein-coupled receptors, the receptor tyrosine kinases, and then you will talk in the lecture 22 about ion channels, and how they perform a receptor function.
So the membrane proteins, first of all, I want to underscore their importance. 50%-- they comprise 50% of drug targets, the receptor tyrosine kinases and the G protein-coupled receptors. The G protein-coupled receptors have this 7 transmembrane helix structure, which spans a membrane. This would be the outside of the cell, and the inside of the cells-- so there's signals going across there.
The receptor tyrosine kinases are another important type of receptor. They are dimeric proteins that in the presence of a ligand dimerize, and then cause intracellular signaling. Once again, they cross the plasma membrane from the outside to the cytosol. And then lastly, there are the ion channels, which also may cross the plasma membrane.
And when you think about these classes of proteins, there's a tremendous amount to be learned with respect to their functions. And they are so important to understand their physical functions in the body, because they really represent the place, the nexus, where signaling happens in the cell.
So I want to briefly show you a picture of a GPCR. It's a 7 transmembrane helix structure. You can see it here. There are about 30% of modern drugs actually target the GPCRs. And here, I'm just going to show you the structure of a GPCR. Those are the 7 transmembrane helices. If you stretch them out, that's about the width of a membrane. That's typical of a signal that would bind to that kind of receptor.
This is a chemokine. It's a small protein receptor. So you can see that structure and how it would go from one side of the membrane to the other. In it's bound state, the chemokine binds to the 7 transmembrane helix receptor through kind of a clamping action. The magenta is the chemokine. The blue and the green space filled parts are actually what holds the chemokine.
And if you look at it where the membrane would be, you can see how you can transduce a signal from one side of the membrane to the other, by the binding of the magenta molecule to the outside of the cell, to those loops outside the cell. That would have a significant perturbation to the biology and chemistry of what's going on on the inside.
So next class, we'll talk about pathways that are initiated by these G protein-coupled receptors, and what that terminology means. OK.