Flash and JavaScript are required for this feature.
Download the video from Internet Archive.
Description: This lecture covers polymer properties, co-polymers, and nature’s polymers.
Instructor: Jeffrey C. Grossman
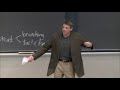
Lecture 34: Introduction to...
It's our last Friday.
Did I hear an, "Oh?" Thank you for that one "oh." It's OK.
I'll take what I can get.
It's our last Friday, and so next week is our last week.
We have class on Monday, and Wednesday will be the last class.
And we've got one more smallish topic to cover after this, which is diffusion, and we'll wrap up on Wednesday.
But today, I want to keep talking about polymers.
So this is our polymer week.
And I want to go back to the properties I've put here, the properties we've talked about.
Right?
So on Monday, we focused on what a polymer is, and we focused on the ways you make it.
All right?
The radical initiation, chain, addition, and the condensation polymers that you know, the condensation where you have two different mers.
And then on Wednesday, we talked about things that matter, kind of like engineering polymers.
Like what are the properties of the polymers that you can change, that we can change?
All right?
And so we talked about, well, you could pick a different monomer.
That will change the properties.
Right?
We've been through that.
You could try to grow longer or shorter strands, so that's going to change properties.
The interactions between the strands, which will, of course, depend on what you pick there.
Right?
And then we talked about the density and the crystallinity and how that could depend on things like cooling rate, or maybe the physical structure itself of the chain.
So what do I mean by that?
Well, when I say physical structure, of course I mean the chemistry that makes that physical structure, so I could branch the chain with the same monomers, but now instead of being one linear chain it's a bunch of branches.
Or maybe you could have a functional group that you can control which side of the chain it's on.
And all those things would lead to differences in density an crystallinity.
And then the last thing we talked about is cross-linking.
And what I'm going to do is I'm going to add one more thing to this list today, but before I do that I want to pick up on the last slide of Wednesday, which is this.
So we tend-- so all this kind of goes in together to give you these solids called polymers.
Right?
But we talked about how sometimes they're not fully solid, and that's what's going to come in the middle.
But you can make a solid thermoplastic, which is these strands.
Right?
So what is a thermal?
Ah, what's the?
Anyway, so with thermoplastic we'll look over here.
There is no cross-linking, so you don't have any of this.
But what do I have?
Well, I have these really long strands that are bonded together with these IMFs, typically in a thermoplastic you would have something like maybe van der Waals, and maybe you have some H bonds.
And these materials, because you didn't cross-link them, and you didn't cross-link them strongly with some kind of strong bond, because of that, you can reheat them and reprocess them.
So these are actually often the plastics that we recycle that we can recycle.
And like I said, they're mostly linear and maybe slightly branched polymers, and they're used in a whole lot of applications, so we call them thermoplastics.
Thermal-- you can heat them.
Right?
You can heat them, and you can see what happens is if I heat these back up, these links can start moving.
They wiggle, and they wiggle until they can just kind of slide past each other and become a vicious liquid and then a melt.
On the other hand, if I've got a thermoset, I heated it up, and then as it set I crossed linked it.
And there are lots of ways you can cross-link.
We talked about some of those.
Right?
And now I've got a pretty high density of cross-links, so that's drawn here.
So I've got these long strands.
Remember, they're super, super long, right?
But now, every so often I have something linking them together.
And if I put a lot of cross-links in, and if they're strong cross-links, then you can get really hard plastics.
Right?
And so these are high-- the thermoset, they're set.
They're set.
Thermoset, good.
It solidifies, and it can't be reheated.
Why?
Think about it.
If I heat that up, then, OK, I might melt the polymers, so the chains are kind of wiggling around, but those cross-links aren't going anywhere.
They're really strong.
And so what happens with thermosets is they're very difficult to recycle.
They're very difficult to recycle because what'll happen is before those things melt the whole thing catches fire.
The whole thing catches fire.
It burns often.
Or maybe it doesn't burn, but when you melt it, you've got this weird chemistry now of the cross-linker mixed into the polymer, and it's not useful anymore.
So these kinds of plastics-- the cross-link is really great.
You make, you know, like I said, over 30% of all toys.
It's used in many, many applications, these harder plastics, but they're not recyclable.
And then, we talked about this intermediate region.
And this is where we ended.
We talked about elastomers, and elastomers are in between.
And so in an elastomer, you've got light cross-linking.
And the cross-linking, like in slime, that cross-linking has a weaker hydrogen bond.
All right?
And remember, we talked about how that could lead to viscoelasticity, really cool stuff.
In other cases, like in the case of the vulcanized rubber-- remember that, Charles Goodyear?
That story from Wednesday?
In that case, you put a covalent bond of sulfur between the strands, and that gave you a much stronger rubber that still had some elasticity.
But now, the best analogy here is still the spaghetti.
I keep coming back to it.
I'm not letting go.
Think about it.
If I've got a bowl of spaghetti, and I pull on it, that's my plastic bag on the left.
All right?
That's my plastic bag.
I pull on it, and those chains are all crumpled up and tangled, and I'm untangling them, and then I'm sliding them past each other, and pulling them until the whole thing rips.
That's the bowl spaghetti.
But now I've got this spaghetti that attaches every so often, one strand to another.
Think about it.
I've got a bowl spaghetti, but in each strand of spaghetti, there's like five places where it's attached to another strand of spaghetti.
Now what happens?
I can pull that as well.
I can pull it because those spaghetti these are all curled up, and so there's some amount of pulling that I can do while they uncurl until those links take over, and I can't pull past them.
All right?
That's what the light cross-linking will do.
And you say, well, if I had heavy cross-linking in the spaghetti, then the whole bowl of spaghetti wouldn't be very easy to move because I'm always up against all the links between them.
All right?
And so that's why these elastomers are so interesting, because they fall-- it's all interesting.
But the elastomers fall in between, where there's some of that uncurling of the polymers until you get to a point where, depending on how much you've cross-linked, and the bonding of the cross-link, you're going to go up against the cross-linking.
All right?
And so these are used, so they're free to move.
Now, must be above its glass transition temperature.
I want to talk about that for a second because I did mention this, and I want to be sure that we all have a good sense of what that means.
And so I wanted to-- taking this elastomer, say, what happens when you melt it?
All right?
And we drew this curve, but I want to go very carefully.
This is the temperature.
Oh, we love this curve, the molar volume.
Right?
And now up here, you've got your liquid.
Right?
We know that.
And then here is the place where it would crystallize.
Can a polymer crystallize?
Sure.
Right.
If a polymer crystallized, then it would just be these strands in a crystal.
What is a crystal?
We know it's a repeating ordered structure.
Yes, polymers can crystallize.
But as we've talked about so often, what happens is because, like we talked about with silica, you know, you've got this very viscous, amorphous, can't find the crystal sites-- same with polymers.
And so you get a glass.
And then this was the Tg.
Now, why am I showing this?
Because with these strands-- and I want to show you.
You know, we've drawn this before where often what you get in polymers is some degree.
It's right here, some degree of crystallinity.
Right?
So what do I do?
How do I do this?
Well, you literally have both.
All right.
So I've drawn this already.
So if I'm here on my polymer, then it might look like what I've drawn already on the board.
Oh, I love doing this.
And then there's the crystal, and then there's more.
I've drawn this already.
Right?
You've got this crystalline region, so you've got the crystal, and here, because I'm below the glass transition temperature, this part here is an amorphous-- oh boy, it's kind of small, but this word says "amorphous"-- solid.
It's a solid.
That part is a solid because it's below the glass transition temperature.
All right?
OK, but now let's start heating this up.
And as I get to the-- so now I'm going to get here.
And what happens is it doesn't actually go straight to the full liquid.
I mean, it's got this crystalline stuff in there, right?
It's got these regions.
This crystal has one melting point.
Remember, the melting point of the crystal is the melting point of the crystal.
It doesn't change.
Glass transition temperature can be tuned.
But this is a melting-- Now, so what happens.
Well, what happens is there's more of a curvyness to this, and you might kind of have the shape look more like this.
So what's happening?
This just gets a little bit more at the detail of how a polymer would melt if it's got any crystalline regions.
And as I've told you, very often it does.
Not always-- it could have no crystalline regions.
It could be totally amorphous, but I've drawn this enough that I wanted to explain this curve with that as our starting point.
All right?
And I've also talked about how the degree of crystallinity is so important for properties, and it can be controlled.
So what's happening?
Well, in this region here-- let's draw that picture.
So now I'm in here.
And what happens is I'm below Tm, but I'm above Tg.
So that means that, literally in the same strand-- this is so cool.
That looks the same.
It's not supposed to be the same because now this is a liquid.
This is a viscous liquid, and this is still a crystal.
That's an xtol.
I'm below Tm.
So the crystalline region has not melted, but the rest of the polymer is above its glass transition.
This is so cool.
This one strand is both a solid and a liquid, one strand.
That's so cool.
That's in here.
All right?
And then I get over here, and now here-- OK, well, let's see.
So now this is all liquid, and the crystalline part is starting to come apart.
So crystal melts, xtol melts.
All right, well, that sort of was supposed to be the remnants of a crystal.
And then above Tm, the whole thing's a liquid, right?
So that's kind of zooming in.
I wanted to make sure that we understood this plot in the context of these polymers.
And that also gives you a sense-- OK, now, that's this one strand.
Now, I've got a bunch of strands.
Did I connect them or not?
And that adds the layer of what an elastomer is.
Right?
Because now you can imagine, is the elastomer above or below Tg?
Where's is Tg?
Are we above or below Tg when we bounce a ball?
Right?
How does that influence the properties of the ball?
A rubber ball is likely cross-linked.
And you can imagine that if you're below Tg, then all that amorphous part of the ball is a solid, so there's an elastic response.
Sure, it could still bounce.
If you're in this region, then that part of the ball is a liquid, but it's likely cross-linked so the ball doesn't fall apart.
Right?
So it's going to bounce differently.
Right?
It's going to bounce differently.
OK, so anyway, I wanted to just go into detail of that to make sure that we feel our oneness.
And I mean, you could add here.
This is, because it's so important in just-- like I was saying, just in terms of what this material, how this material behaves, you could add the glass transition temperature, which, as we've already talked about, has a number of ways that it can be tuned.
On the other hand, it may also be tuned by some of these other things that you do, but it's a very important part of the polymer property ecosystem.
OK.
Good.
So that's the elastomer.
Now, there's one more thing that we can do that I wanted to talk about.
Oh, that's the picture.
Yeah, I thought I'd show you another picture.
So I'm not the only one that draws things like this.
All right?
OK, there's a nice one from Encyclopedia Britannica, and you can imagine this region being a solid down here, then a liquid.
But that's still solid, and then that region melts all at once at Tm.
The last thing that I want to add is another way that we have to control polymer properties, and it's another bullet here, and it'll be our last one.
And it's really the composition and sequence.
So what do I mean by that?
Well, it turns out that-- remember when we did condensation.
Right?
Polymerization, a poly condensation it's called.
The condensation polymerization.
We had two different mers.
And we could have picked the box.
Remember, the box inside the mer could be different things.
And then when we've done the radical initiation, we've just had one mer with the double bond.
Right?
Now, if you have one mer, then that's called a homopolymer, Homopoly.
And if you have two, it's called a copolymer.
The thing is that we actually can even make copolymers with both approaches.
And not only that, but we're learning more and more how to control the sequence, and that's what this shows here.
So I could have a polymer A.
Let's say I have a-- yeah, I'll put it below this.
Let's say I have a, you know, polymer, polyethylene, PE, which is-- oh, we know now how to draw these-- C, C, N. And then I've got the other one, which is PVC polyvinyl chloride, and that looks like this-- C-- all I've done here is swap out a hydrogen for a chlorine, and I've got a totally different polymer.
All right?
Some other end, OK?
And an N, whatever.
I can now alternate these.
I can alternate them, and I could alternate them in this way.
So PE might be my A, and PVC might be my B.
And if I alternate them, we might-- you know, if it's like regular alternating, we might write PE-- this is how we'd write the copolymer-- a, PVC.
All right, but you could also have it be random, so then you'd write PE-- you can take a guess-- r, PVC.
Right?
You could write a grafted version-- PE, g-- well, you get the point-- PVC, and so forth.
What I mean when I write this is that I've taken these two polymers, and I've copolymerized.
I mean, sorry, these two.
Well yeah, these are the polymers.
I'm taking the monomers, and I've made one polymer out of the two of them, but I've controlled the sequence.
It's not-- Now, maybe it's not controlled.
Maybe it's random right there.
Or maybe I've figured out how to do this in a way that I have the backbone all one type, but I can have these side chains, the branches, another type.
That's really powerful, it turns out.
Or maybe you can control that I have a certain number of them, and then a certain number of the other, and this is called a block copolymer.
And some of you may have heard of block copolymer chemistry, which is growing, is a very growing, very powerful field.
Because when you make these blocks and you control their properties, you can imagine that you control all sorts of things about how that polymer behaves.
Imagine you make something that bonds to A but not B.
Like dissolves like.
All right, we've been there.
B is something that bonds to B but not A. OK, what's going to happen?
They're stuck, but they're going to try to come together and stay apart in the same strand.
All sorts of interesting things can happen.
It can be engineered when you can control these blocks or these graphs.
All right?
So that's the last thing that we-- Here's an example.
This is-- I was trying to look for a good exam-- here's something called-- actually, what is it called?
Surlyn.
Who comes up with that?
I don't know why that's the name, but that's the name.
It's the Surlyn resin, which is the ingredient that goes into the polymer, OK?
And look at what it does.
Oh, it provides clarity, toughness, versatility.
Surlyn is a leading choice for food, cosmetic, medical device, skin, stretch, packaging, as well as golf balls.
The tunability is enor-- why?
What is Surlyn?
Well, we don't know exactly because they won't tell us, but Surlyn is actually really cool.
It's using-- OK, it's using-- where?
Dah!
Where did I put it?
Composition, sequence-- it's using sequence to make a graft polymer where one type is going along the chain and the other type is coming out.
But really importantly, the other type is made specifically to form ionic bonds.
So here B is made so that it can form ionic bonds with other parts.
Ionic, remember, that's basically by grafting, by creating a copolymer, where one type wants a form ionic bonds.
I've cross-linked.
I've made a cross-link built into the polymer itself.
The cross-link is going to be linking the strands together, maybe within itself, maybe with other strands but with an ionic bond.
Right?
But I've done it all just built into the polymer.
And that's why you can see here.
Look at this it's an ethylene copolymer.
All right?
So ethylene is the backbone.
The co is what's coming out of the side, and that's an ionic bond, so it's also called an ionomer.
What's an ionomer?
A polymer that has ionic cross-links, ionic bonding cross-links.
And what's cool about that?
Well, you get the whole world of ionic bonding now in the mix.
And so what does that mean?
Well, you could have it be very strong, golf ball.
You can have it be thermally responsive.
Right?
You can tune at what temperature these bonds break.
There's all sorts of flexibility, and you can see it right here in how they're marketing it.
It can do almost anything.
Another example of the copolymer-- I'll give you a couple examples of copolymers.
This is one that you may have seen, maybe many of you experienced, you just don't remember.
This is the diaper.
Right?
And so what what a diaper is is it's got this copolymer made out of acrylic acid.
These are the monomers.
Notice the double bonds?
Oh, we're going to take advantage of those, and we're going to make a polymer, but we're going to do it in a controllable way.
So we're going to take these two mers, and we're going to control how they come together.
And very importantly in these kinds of materials-- they're broadly called hydrogels.
These are materials that can absorb hundreds of times by weight, hundreds of times water into them.
Why?
Because in one of the copolymers you've got this sodium atom, and what happens is there's the dry state.
It's all curled up and the chains want to be all together, but as soon as you introduce water, those sodiums like going into the water.
And what happens?
They leave behind this oxygen that really wants the water because it's a negative charge.
And so you get a sodium ion going in the solution.
You get the solution coming to the anion .
And even more than that, now if the sodium leaves, then what happens?
Well, what happens is all those negative charges are left and they repel each other.
That's going to help this whole thing want to expand.
Right?
So that's a diaper, 2% of all landfill by the way.
Now, so that's another example of a copolymer and a really interesting way of tuning the properties.
In here it has to do with, what did we do?
We tuned the ionic character of the backbone.
We tuned the charge.
We made it responsive it in terms of its charge to something, in this case water, the presence of water.
That's cool.
OK.
Last one, and here I want to talk about mechanical strength.
So this is the tensile strength.
So how far could you pull this thing, this material before it breaks?
And then here's the elongation until it breaks, so it gives you a sense.
Now the tensile strength, this is how far can you pull it in the elastic regime?
You can see that for steel it's really high, but you can't pull it very far before it breaks.
We know that, right?
So steel is really strong, but it's not very flexible.
There is nylon.
We did nylon, so it's kind of not nearly as strong, but it has a lot of elongation.
There's fiberglass.
This is plastic reinforced glass, where you can get a lot of strength, very little flexibility, more than steel, a little bit more than steel.
Well actually, it was a lot more, but still it's only 3% or 4% elongation, and here's cellophane.
That can be a naturally occurring polymer.
You can also make it synthetically in the mix.
And I want to point out nitrile.
Nitrile rubber is a really interesting copolymer, and many of you may have seen or used-- if you're in a lab, you might use nitrile rubber gloves.
You might have them at home at the sink.
And one of the advantages of nitrile rubber is, again, this enormous tunability by just picking how you copolymerized.
How much of one did you put in the other?
And this is-- maybe I'll use this board here because it's a really cool copolymer, where you're putting in acrylonitrile.
So here's what this looks like.
So you put your CH2, your CH, your double bond your CH, your CH2, and there it is.
And that's going to repeat some number of units that you control because it's a block copolymer before you put this one in, and here's the kick.
It's got a triple bond nitrogen on it.
And that's going to go a certain m.
All right?
Now, that comes from acrylonitrile and butadiene, but again, it's the synthesis to make the copolymer.
It's the sequence.
I keep on forgetting where.
It's up there.
It's the composition, the architecture.
How did I put those copoly-- if you do this in the right way, it's the amount of the acrylonitrile that you put in will control the strength.
It'll make it stronger and stronger, because you've got this really nice, strong bond in here, and this will give the material a lot of strength.
So that's why you can go up to, you know, fairly reasonable.
But then, how much of this I put in is going to determine how far you can stretch it, and so you balance those.
And you can make a whole bunch of kinds of nitrile rubber.
OK, so I hope this has given you some examples of the tunability of polymers.
And you know, if you take the bigger picture-- and this is a little hard to read.
You know, it will be in the slides, so you can look up this paper here.
It was published in Science a few years ago-- and you look at the fracture toughness with the yield strength.
So what is that?
Well yield strength is-- Remember, we know that because it's when you're in the elastic region, and you're pulling, and then you yield to plastic deformation.
Right?
That's the yield strength.
Now what about fracture toughness?
Well, that's if you start a crack, does the thing tend to crack more or not?
That's the fracture toughness basically, right?
And so you could put materials down on this plot.
You've got ceramics.
You've got concrete down here.
You've got-- so you're not going to be.
So the polymers sit here.
All right?
Here's metals, and alloys, metallic gases.
You can look at this on your own, and look at each one of those, but I just want to point out, polymers have a fairly wide range, but there's so much interest in going beyond.
There's a lot of interest in using polymers in many other applications.
We can't get there yet because we can't push it out here or maybe out here.
We still need to figure out how to tune it more.
We've got all these ways to control the properties, and we're still only at the very beginning of understanding how to engineer polymers.
Now, there is no better way to make that point clear than to look at nature.
All right?
And I already showed you the tree and you know, the examples of nature as a polymer engineer.
I want to talk about that a little more because nature is not just a polymer engineer.
Nature-- I'm going to write this down nature.
Nature is a polymer engineer gone wild, and I'll show you why.
Polymer engineer.
Humans, what can humans do?
Well, we are a natural polymer engineer material, but what can we make with all of what I've just shown you?
What can we make?
I can put like one, or maybe two, or if you really go into the research, you get three mers, trying to control where they are in the chain.
All right?
You've got three, maybe two.
We have really two in most materials.
Copolymer-- we're so proud of this nitrile.
Two mers, and we control them, and we make rubber sheets.
But nature-- but then they're everywhere.
They're everywhere is just that.
All right?
So nature can have-- so humans have the same functional group every stop.
All right?
OK, that's one mer.
Maybe I've got two, maybe two or three.
Nature can have different groups, and here's the key.
It can have them everywhere.
OK?
So what I have is I have many, many more possibilities.
I mean, we have these possibilities too, we just can't control it.
All right?
So if I look at-- like let's go back to condensation polymerization because this is what nature does.
So this was what I drew for you on Monday.
There's a dicarboxylic acid.
Here we're making nylon.
And a diamine, and we're making polyamide.
And remember, the box-- in nylon 66, the box is 6 carbon atoms.
I call that boring.
It's at six carbon atoms from hydrogen, but that's kind of boring.
But in nature, this box is an amino acid.
That's much more interesting.
That's much more interesting because if you look at an amino acid, and this is an amino acid-- why is it an amino acid?
Well, it's got an amine group here and a carboxylic acid there, so it's an amino acid.
But see, here's the thing, R.
This is nature's "the box." We can put six carbons in.
We're really proud of ourselves.
Nature can put almost anything it wants for R. All right?
So R, just to spell this out, is nature's choice, and I'll show you what it chooses.
Nature's choice-- because there are hundreds of amino acids, and this R group can do many things, but just 20 is all we need to make proteins.
Most proteins are made out of just essentially 20.
But if you do the math-- I think I have the math here-- and you take-- let's see.
OK, if I take two amino acids.
Let's say I take two amino acids, so I've got two different R's.
Two amino acids, and I've got a length is 2, and this is called the dipeptide.
I'll tell you why in a sec.
OK, but let's compare this now.
Now I've got 20 amino acids, and I just told you that 20 is what nature makes most proteins out of, 20 different R's.
There's an amino acid.
R is nature's choice.
But see, if I've got 20, then I've got 20 squared equals 400 dipeptides.
I've made a two-unit polymer.
It's not a polymer.
It's a peptide.
But now, what if I take the 20, and I've got-- but now I've got, let's say, 1,000 units long, then I would have 20 to the 1,000 possibilities, which is 10 to the 1,300 combinations.
So 10 to the 1,300 possibilities-- that's because there's 1,000 units, and I've got 20 amino acids, right?
But now you think, how do you possibly think about what to do?
That is nature.
It's had 1 billion years, and it's given us the world that we live in, that we know.
It's messed with these combinations in a way that's impossible for us to even understand.
That's why I say, nature is a polymer engineer gone wild.
It's got almost limitless flexibility, and it's used it.
Right?
Now, how does nature make its polymer?
Well, its condensation polymerization.
This is just what we saw.
Look at that.
This is what an amino acid is.
It's got this carboxylic, so there's the OH group that can condense with the H here and form the link.
There it is.
That's called a peptide bond.
When two amino acids come together, that's a CN.
That's a peptide bond.
Right?
But look, this had R1.
That had R2, and I've got 20 different amino acids to choose from.
The possibilities are endless.
So the protein synthesis is condensation pol-- you knew that.
You knew it couldn't be anything else because there is no double bond.
Where would I have done a radical initiation with these amino acids?
If I'm nature, I've got to make it with condensation polymerization.
That's how we are made.
OK, now, so just to give you a sense, so the R-- I'm not going to go through this, obviously.
I'm just giving this to you as if you want to read more about it.
There's some wonderful charts here, 20 amino acids, the 20 that are most common and that nature puts together.
And what they've done that's really nice here is they've grouped them, right?
Because what did nature choose to do?
How is it most utilizing the properties, the tunability in these amino acids.
I said there were 20, but most proteins are-- I'd say there are hundreds, but most proteins are made from these 20.
It gives you the tunability in having maybe non-polar groups.
Right?
So it might be then hydrophobic.
Polar groups, hydrophilic-- maybe you can put, nature can put, R groups in there that maybe have a charge, or that lose or take an ion.
All right, so it can play with the charge, and it has done all of that.
It can have groups that become acids, that make something acidic, and so forth.
OK, so that's cool.
What has nature done?
Well, I got to show you this spider because-- and if you're interested, Professor Bueller does some wonderful work on spider silk-- because I think this is a great example of something that nature can do that gives us a sense of how far away we are.
Like I said, we're very proud of this, and we want to do more.
And so we take our two mers, and we mix them together, and we make branches, and maybe we're going to try to add a third.
Meanwhile, nature has had a lot more flexibility and a lot more time.
What can it do?
Well, here's spider silk.
Now, this is a spider.
Spider is an incredible polymer synthesis machine.
It's an incredible polymer synthesis machine.
And here it is weaving a web, and I love this-- [MUSIC PLAYING]
--because I think it's so cool.
OK, there's music, I guess.
I forgot about that, and there it is.
Now watch.
Out of here, this is the back of the spider.
There it is right there.
It's making protein.
That's called spider silk, but these are proteins.
These are polymers.
It is doing condensation polymerization right there, and then there's all sorts of structural stuff that it does.
Right?
It's got a specialized hook.
It knows how to step.
It creates-- look at that.
There's a branch place where it knows to put it.
Right?
It's already created the glue.
So not only is it putting this spider silk out there, but it can put other types of polymer depending on what it needs.
Does it need something really sticky, less sticky?
Right?
And so there it is weaving its web, and it's generating this polymer on the fly.
It's doing condensation polymerization.
Now a couple properties about spider silk.
OK, so here we go.
Let's see.
So spider silk, this is just one example of what nature can do.
It's five times stronger than steel.
Remember the mechanical strength chart, nothing was stronger than steel.
Spider silk is five times stronger.
Just to give you sense, the example, I found that I like.
If you had a spider silk that was a pencil width, and you made a strand, it would stop a Boeing 747 in midair.
That's how strong it is.
Oh, it keeps strength-- here's another thing-- below 40 degrees C. We can't do that.
Just take a rubber ball and put it at that low of a temperature and try to bounce it.
It's going to shatter.
Right?
Spider silk can keep that strength and not break.
Its elastic, so it's got-- throughout all of this, it's got an elastic property of 4x, so it can be stretched to four times its original strength.
Compare that with nitrile.
Nitrile rubber could also go to very, very high elastic elongation.
All right?
So if you go back to nitrile-- here it is in the table.
We're very happy with this, but this only had two monomers to play with.
Right?
We played with two monomers, and we got to here, and look at the sacrifice in the strength.
Look at how much we had to sacrifice strength.
Spiders don't have to do that.
OK, and oh, here's the last one.
Fully recycles-- now the thing is that this is actually kind of incredible.
Spider webs get dusty.
They lose their stickiness.
So many spiders know this and just simply have to weave a new web every day, but they don't leave the old web there.
They actually eat it, and they fully recycle it, fully.
Right?
They eat the web, fully recycle it, process it, have this condensation polymerization work, and make a new one the next day.
All right?
So we don't come close to this spider.
We don't even come anywhere near it in terms of where we are.
Even though I gave you all these wonderful things that we're doing with engineering, we still have so far that we could go if we could just figure out how nature works.
OK?
And this gets me to what we do, and so I've got my "why this matters" now.
And so a spider eats its web, fully recycles it, spins a new web the next day.
Here's what we do with our polymers.
I already talked about the oceans.
Here's what we do on land.
These are tires.
Now, tires are very difficult to recycle.
Why?
Because they're too vulcanized.
They've got too much cross-link.
Remember, if the cross-link density is too strong, which you need to make a tire, then you can't recycle it.
And in fact, what happens is-- and there's a tire mound.
Here's it is from a satellite picture.
Those are tires.
Those are tire mounds.
Here's what happens when one catches fire.
Here's what happens when many catch fire.
All right?
It's actually a very hard fire to control once they catch.
But we don't really know how to recycle them well yet.
What can we do?
On the science and engineering side, the fact of the matter is there is a lot of work to do, but there are promising directions.
So I wanted to leave you with a little bit of that, and I'm not going to go into great detail.
I just want to show you, and there's references here you can look.
This was published a couple of years ago in Nature.
One direction is in self-healing polymers.
This is a very exciting direction.
What can we do?
Well, we can go away from this whole single-use idea and make stuff last longer.
All right?
So that would be beneficial.
Maybe not if it ends up in the ocean, but in terms of just how long we can use these materials.
So one direction is, well, you've got these polymer networks, and you incorporate little gels, little beads in here, but the beads don't open up until a crack comes along.
So they're sensitive to a crack.
And when they feel a crack in the material, they open up, and they pour a healing liquid that then solidifies.
That's a self-healing kind of approach.
You could do that at different scales, all the way down to the strand.
You can do that on larger scales.
And here's a whole system where there's actually this healing material being flowed through a polymer structure, just like arteries in our body.
Again, always there to try to heal the material.
Right?
Another direction is in fully recovering.
And again, I don't want to go into full detail here.
You can look up some of this stuff.
This was published last year.
Can we take the polymer and chemically decompose it all the way back to the monomer?
Can we go back to where we started?
Can we do what the spider does?
Right?
The answer is no, not today.
But if we could, that would open up a whole lot of doors for recycling that are closed today.
Can we do this in a way that is efficient?
Right?
And then, another direction of work that I think is very important is in making thermosets so heavily cross-linked so you get all the hardness and all the properties you need of the polymer that's heavily cross-linked, but easily breakable in the cross link.
And there is good work going on in this direction.
Can we make degradable thermosets?
That's another really important direction.
And last, we should be encouraging people, if you can't do any of this, at least take the polymer out of the landfill and make something with it.
And there are projects-- you can look at here, Waste Management Journal, in making bricks out of polymers, incorporating them into construction materials.
Right?
These are important directions, and we need a lot more hopefully in the very near term.
hopefully in the very near term.
No more just talking about alignment.
OK, have a good weekend.