Flash and JavaScript are required for this feature.
Download the video from Internet Archive.
Description: This lecture discusses the ground-breaking experiments that brought the scientific community closer to understanding the structure of the atom.
Instructor: Jeffrey C. Grossman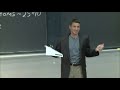
Lecture 3: Atomic Models
Welcome to the third lecture of 3091.
Let's get started.
Today is a very exciting day, because we are going to discover the electron together.
That's our goal today.
It's right there, discovery of the electron.
And we're going to start talking about models of the atom.
Before we do that, I want to count.
I want to count, because that's what we did on Friday.
And we'll use this problem actually twice today.
Either way, like if I do a problem in class, and it's in a goody bag and stuff, I don't know, it might be the kind of thing you might be asked on a quiz.
I'm just saying.
So that's good to know.
If I ask you this question, because we counted on Friday, we learned how to count.
And we learned about the mole and Avogadro's number and how that gets us into and out of the atomic world, right?
So if I say, how many gallium atoms are this strip of gallium?
Well, we can do that now, right?
Because well, how do you start?
This is where you start.
And you are all taking it with you everywhere now.
Remember on Friday, we talked about how you got to bring it with you everywhere and tweet about it and send me pictures and all that.
I need the density, right?
I need the density.
Density in the periodic table.
Let's start with the density.
So if I have the density of gallium and I looked that up as 5.9 grams per centimeter cubed.
OK, that's good.
Well, you know, from the strip I can measure it with the super most accurate ruler in the world that you have now in your hands from the goody bag.
And you see those are the measurements.
And so if you measure it, you have a volume.
OK, it's those three numbers multiplied.
I won't write it out.
It's 0.5 centimeters cubed.
And so now with the density and the volume, I get that that strip has 2.95 grams.
OK?
Now that sounds pretty good.
It's promising, but I want the number of atoms, right?
I want the number of atoms.
And that's where, again, you go to here, because in there is the grams per mole and also the atomic mass units, which, as we talked about Friday, are the same thing.
Right?
And so if I look that up, I have that for gallium, we have 69.723 grams per mole, right?
And so that means that in 2.95 grams, I have 2.95 divided by that number, 69.723-- let's raise this up a little-- which equals 0.0423 moles.
I still have not answered the question because the question says, how many atoms?
Right?
But that's where n sub a, or the mole, or this dozen, right?
But it's not a dozen.
It's a much bigger number.
But this number, Avogadro's number, comes in.
And so in that strip of gallium-- ah!
No.
In that strip of gallium, you've got, in the GA strip, you've got 0.0423 times na atoms.
That's something like, you know, 2.5 times 10 to the 22nd atoms.
That's a lot of atoms in that strip, but I was able to do.
But the secret is the-- right, remember?
One atomic mass unit gets you back and forth between one gram per mole.
And by the way, the atomic mass unit is nothing.
It's just a mass unit.
I mean, one AMU happens to be, by the way, oh, roughly 1.66 times 10 to the minus 27th kilograms.
Huh!
That works because now you can multiply this by a whole mole, and it's a gram.
Back and forth, OK?
Good counting.
Starting with counting.
We're going to end with counting.
But in between, we're going to discover something beautiful, because last week-- OK, the thesis of this whole class, remember, is electronic structure holds the key to everything.
Everything in your life can be understood if you understood the electronic structure of the elements.
Week one, last week, we talked about this.
We built it on Friday.
We talked about elements.
We did a few reactions.
We talked about balancing.
We made sure we have enough nitrogen to grow plants for 100 million more years, eliminate reagents.
This week, we're going to answer the question, why are these things different?
We're switching gears, right?
We know that there are these indivisible atoms now.
And we built a whole table of them, right?
Mendeleev.
But now we want to know why they're different from one another.
What causes that difference?
And that starts with the discovery of the electron.
And really this is such-- so let's write this here.
We'll have this board.
This is such a great story.
This is a detective story that starts with an atom looking-- so these are atom models, OK?
So back in, oh, let's say, 18-- ah, OK, 460.
If it's Democritus, it's 460 B.C.
And then remember, we sort of went all the way to Dalton, who, in 1803, he said, yup, Democritus, we're going with that.
We're going with that.
And oh, there are these indivisible things called atoms.
So that was 1803.
But we need to understand them in order to explain this.
We need to understand them.
And so this is a detective story.
It's one of the greatest ones ever, ever, ever played out in science.
It led to nothing less than the understanding of all of nature.
OK, now, how did it start?
Well, it started with J.J. Thomson.
Now, so J.J. Thomson-- by the way, he won the Nobel Prize for this discovery.
He's credited with discovering the electron.
Also seven of his students won the Nobel Prize.
That's cool!
And I remind my graduate students of that all the time, without trying to put any pressure on them.
How did he do it?
Well, you see, he had these things.
There is his lab.
There's Thomson, there's his lab.
And these things were around.
They're called cathode ray tubes.
Cathode ray tubes.
What do they do?
All right?
What do they do?
Well, your grandparents would know something about them.
I'll talk about that in a few minutes.
But Thomson, Thomson was interested in seeing what he saw on this thing here.
And that's the photographic plate.
So what we're going to do is we're just going to draw the cathode ray tube, draw it as best I can here.
So there is a cathode ray tube, and over here we've got a cathode, and over here we've got an anode.
So this would be a cathode, and this here is an anode.
OK, good.
Now, that means that if I hook the other two pieces of metal, two pieces of metal, and if I hook them up to a voltage supply, then I can get charge on them.
We know that already.
But what they didn't understand is what happened next.
You see, because if you crank the voltage up really high and you put a phosphor screen here-- so this was a phosphor screen-- and if you crank the voltage up high enough, and this is what Thomson did, you pump the gas out of it.
Right?
So you get it so there's not too much stuff in there.
Then what happens is something shot across and lit up the screen.
Something.
What?
They really didn't know, and when you don't know, you experiment and you apply the scientific method.
And one of the things that Thomson did is he said, well, what if I put some charge plates here, like this and this?
So I have a [? set, ?] right?
So it is said, this is going to have charge that flies off.
We know that, because we kind of know what happens.
But he didn't know what was happening.
He's just cranking the voltage up, and all of a sudden the screen lights up.
So he's like, well, let me put some charge plates here and see what happens.
And what happened there is it went like this, right?
And it struck the phosphor screen there, and it glowed there.
So that's with charge, with charge plates.
Right?
No charge plates, just to be perfectly clear.
And he could play with this, right?
And people do play with this, to my great surprise, if you go onto the internet and you Google this.
You find people make these and play with them, and that's a magnet.
This is what it looks like, right?
But we didn't know what this was, right?
So that's a magnet, and this person is just turning over whether which part of the magnet, where the magnetic field is oriented.
And you can see, you can really mess with this.
What could it be?
It's responding to an external field.
It's responding to an external field, so we know right away, this tells us that atoms are not the ultimate form of matter.
Atoms were not the ultimate form of matter.
That was a big deal, because the atoms here were neutral.
They didn't respond, so this must be something else that's flying off and responding, that I can see visually on this photographic plate.
It must be something else.
Well, he was also-- Thomson was-- oh, ha!
Going down here, I'm going to use this so it's right next to it.
He was not able to measure the mass of these things, but he was able, using a combination of magnetic and electric fields and the little bit of Maxwell, some ENM, he was able to deduce the charge to mass ratio.
So this falls over here.
I'll put it here, from Thomson.
So he was able to get the charge to mass ratio.
And he was able to measure that at minus 1.76 times 10 to the 11th coulombs per kilogram.
Now, he knew it was negatively charged because of this, right?
He put the plates on, and it wanted to avoid the negative plate.
So they knew their ENM at the time.
They knew that the negative charges would repel.
So he knew that it was a negative charge.
Now, the last thing, oh, is this one important for chemistry!
He would swap in different materials, different metals for the cathode and anode.
For the cathode especially.
And the results were the same.
The results were the same.
Oh!
Independent, independent of metal.
Independent of the metal.
That meant this thing that he was observing for the first time was fundamental.
There's something fundamental about it.
Fundamental!
This was a really big deal.
This was a really big deal.
And it really opened up the idea that the atom had something else in it.
Now, see, Thomson knew that the atom was neutral, like I said.
So you've got these charged things coming off of these atoms, which are neutral.
That means that it must consist of these charged particles.
But if it's neutral, it's got to have the other charge of particle in it as well.
And so the detective story goes on.
So this would be Thomson around 18-- oh, things really picked up.
1807.
And he said, well, OK, these electrons must be inside of the atom.
That's how I'm liberating them.
Crank up the voltage, and they must be in there.
But so must positive charges.
So must positive charges, OK?
And that was Thomson's model.
So at that time, you could really have this picture of stuff inside the atom.
Now, like I said, he couldn't measure-- they didn't have a scale good enough to measure the mass of the electron.
He could get it out, all right?
But it was Millikan and his very famous experiments called the oil drop experiments that gave us the actual charge.
And then from this ratio, we can get the mass of this mystery thing that was coming out of atoms, OK?
And instead of trying to draw the Millikan experiment, I found a wonderful, short, like one-ish minute video, which I'll play, because it shows how the Millikan experiment worked.
It's really cool.
Oh!
[VIDEO PLAYBACK]
- Robert Millikan, working at the University of Chicago, succeeded in measuring the charge on the electron.
That's not happening.
- He allowed the fine spray of oil to settle through a hole, into a chamber where he could observe their fall.
The top and bottom of the chamber consisted of electrically charged plates.
He introduced a source of x-rays which can cause creation of charges when they strike matter.
Charges reduced by the x-rays attached to an oil droplet, and producing one or more charges on the droplet.
When there is no voltage of light, the fall of the droplets is determined by the mass and the viscosity of air through which they fall.
When a voltage is applied, the droplets that have a negative charge will fall more slowly, stop falling, or even rise, depending on the number of charges on them.
By adjusting the applied voltage and observing the droplets, both with voltage off and voltage on, Millikan was able to determine that the charges on the droplets were all multiples on a smallest value, 1.6 times 10 to the minus 19th Coulombs.
He took this to be the charge on a single electron.
[END PLAYBACK]
All right, so now you see that that would have been-- I hope that was-- could you hear that in the back?
Sort of, OK.
All right.
Oh, I got this.
You know, that would have been hard for me to draw the animation, but what a brilliant experiment, right?
So spray some oil in a container, OK?
Try to get the drop small.
You don't know how small they're going to be.
And then as they fall-- OK, have an electric field inside there.
And then as they fall, zip them, zap them to charge them.
You also don't know how much charge you're putting on them, right?
But you're charging them.
And if they're charged and they're in a field, and there are these tiny, little microscopic droplets of oil, they're going to maybe slow down or suspend or maybe even go the other way.
And what he observed by doing this over and over again, that there was some multiple that you never got below, right?
And you couldn't say necessarily for 100% certainty that it was the fundamental charge of electrons.
It might have been a multiple of that.
But it was pretty clear.
You could never get below that.
So that was the charge discovery in this detective story, and it allowed us to understand that the electron had a mass and it had a charge.
And that we knew both of them at the time.
So at the time then, we already had a much deeper understanding, if you think about it, just four years later than the time of Dalton because of these two experiments.
Yeah?
So we said that Thomson was 56-- he'd be [INAUDIBLE] 1940s, so wouldn't that be 1907?
1856 to huh?
Thomson was alive.
Alive.
[INAUDIBLE]
I think I read in my-- he was ahead of his time.
[LAUGHTER]
Thank you very much.
I think I read this wrong, and that's because I don't have my glasses on.
So now that I look at that, it wasn't that small of a distance.
[LAUGHTER]
Thank you very much.
You make mistakes.
You make mistakes, and that is how you learn!
[LAUGHTER]
You don't make progress.
Progress has almost nothing to do with success.
I mean this.
I'm using my own mistake here as an example.
Progress has almost nothing to do with success.
Progress has only to do with what you choose to do with failure.
Did somebody just say, whoa?
Thank you.
Thank you.
That hit me here.
All right.
OK, let's do a why this matters.
Let's do a why this matters.
Why does this matter?
Well, because, see, these guys were trying to figure out what was inside of an atom.
But other people, like John Baird, said, well, wait a second.
You just gave me a paintbrush.
You gave me a paintbrush.
Look at this.
That's a paintbrush, painting with a magnet.
It really is.
And the screen would light up over here.
And so he said, well, I can paint.
I can paint pictures.
And this really is the first television screen.
This was the first TV screen, and all they needed to do, there-- OK, right?
There it is.
Look!
That's a cathode ray tube.
Maybe that's one of Thomson's students who won a Nobel Prize.
And but now, you put this down, and you put these sort of things around it.
What are those things?
Magnetic fields, that's all it is.
It's just magnetic fields.
It's Thomson's experiments, right?
But now they're using it to zip the beam around faster than you're [INAUDIBLE] keep up with so that it looks like a picture.
Now, electron painting had never been done before, because we didn't know that we had these electrons.
But as soon as we knew, boy, did that launch a completely new era of screens.
Right?
The era of screens.
We don't use cathode ray tubes.
I was going to say, ask your grandparents.
They'll tell you about the cathode ray tube TVs, which they all had.
But you know, we don't use cathode ray tubes to paint with electrons today in that way, but we still paint with electrons today.
Right?
Youre OLED screen is still simply an electron-based painting tool, right?
OK, we're just pumping the electrons into the phosphor in a different way, and we'll be talking about that as we go through the rest of this week and we understand how electrons interact with light coming in and out of an atom.
OK, so that's my why this matters.
And by the way, a side note here is that when TVs first came along, green was pretty easy.
There were a lot of chemistries that were used for this screen.
You put a different chemistry here, and it lights up differently when electrons hit it.
Why?
Wait until Wednesday.
Green was easy.
Yellow was easy.
Red was hard.
Red was hard.
They couldn't get a good red.
And of course, that's essentially the reason there were no color TVs until the '60s, right?
And the answer, of course, was here.
The answer was that there was a phosphor that worked, but it was yttrium orthovanadate with a little bit of europium added to it.
Just a little bit of europium.
Why did that work?
Again, we need to understand how electrons interact with matter, which is where we're going.
And speaking of yttrium, speaking of yttrium, this is also a side story, but it's kind of worth noting.
You know, elements are named often-- well, elements can be named after many things, right?
In this case, it was named after Ytterby, Sweden.
Ytterby, Sweden, is a pretty cool place.
They had this one cave.
You got to go to it.
It's really cool, because four elements of the periodic table were all discovered from Ytterby, Sweden, in this one cave.
Four!
And I keep thinking, where is that cave in Cambridge?
[LAUGHTER]
Hm?
They cannot discover any more elements, because there's no other way to mess with the name Ytterby.
[LAUGHTER]
That's pretty cool.
That's pretty cool.
That's a cool cave.
That's worth visiting.
All right, so the structure of the atom in the 1900s-- thank you for the date correction.
1900, the structure of the atom was as follows, all right?
The atom is electrically neutral, but there are these negative charges.
These negative charges called electrons.
The electron has a very small mass.
We know that from the oil drop experiments, right?
Which means that the bulk of the atom is positive, because the bulk of the mass of the atom-- you know, the atom weighs one thing.
The electron is just a small part of that in terms of the mass, so most of the mass is positive.
And there was a question.
OK?
So we're getting somewhere, but how do these charges really look?
How do these charges arrange inside of this atom?
Is Thomson really right?
And that was the next part of our detective story, was to really understand what these charges are doing inside the atom.
I'm carrying this around like my security blanket, which is making me feel very secure right now.
And you should all carry it too.
This part of the story relies on another type of energy that was being discovered at the time.
And you all may recognize this as radiation.
Now, radioactivity, radioactivity is nothing more than ray activity.
This is what they named it after, ray activity.
That's radioactivity.
And it was these three people who really pioneered the understanding-- gesundheit-- of radioactivity and in particular, the fact that some elements seemed to just be radioactive.
What did that mean?
Well, it means they gave off these rays of energy.
That means they gave off these rays of energy.
You had Henri Becquerel.
You had Marie Curie and Pierre Curie.
Notice I didn't even try.
Where's Jerome?
I didn't even try with the French.
But anyway, they took these materials, like uranium ore, minerals made out of uranium, and they said, this stuff looks like it's got something coming off of it.
Let's see if we can find what elements are causing that.
All right?
And Marie Curie, she found polonium and radium this way, those two elements.
Polonium named after her home country, her native country of Poland.
And this stuff gave off all this energy, and it was so energetic they could literally put it in a cup and have some writing there and put it in a super-dark room, and it would illuminate the picture.
Right?
It would illuminate the picture.
But this is not a recommended way to light your pictures.
So Pierre carried a vial of radium, because it was so cool and it glowed!
He was like, look at this stuff!
Right?
And he actually did have bouts of radiation poisoning, although he died, tragically, when he was struck by a horse-drawn carriage in 1906.
Marie lived much longer and won two-- but also died because of radiation poisoning.
But she won two Nobel prizes.
First woman to win a Nobel Prize, only woman to win two, and for only person ever to win one in two different disciplines, physics and chemistry.
She was quite a brilliant scientist.
And they gave us this radiation.
Now, OK, but see, OK, they were really into radiation, but it was Rutherford who said, well, wait a second.
Maybe I can use this stuff to keep going along this story.
Right?
Rutherford was like, well, maybe there's something we could do with this radiation.
And so what happened?
Oh, there it is!
And so I'm going to put it right above it so you can see.
So he said, well, OK, if I take something that glows, maybe some radium, and I carve out a little thing here, and maybe I put some plates to kind of protect scatter, I can make a beam of this stuff, OK?
So this would be like maybe radium, let's say.
And then what he did is he took this photographic plate and he curved it, just so he could really collect as much as you could, right?
So you can really collect at really high angles.
And he did the same thing.
He put charged plates on either side, and he found that you had three different types of beams.
He had one that went like this, one that went like this, and one that went like this.
And we now know that this is beta, this is gamma, and that's alpha.
Let's put this right above.
This is so cool to watch.
So what Rutherford did is kind of like this.
He had stuff and he made a beam of it, and then he put stuff around it.
But if you got a beam of something that might be charged, you're not going to smash it or burn it.
You're going to put some plates around it, or a magnetic field or electric field.
And that's what he did.
And notice, alpha bends down, towards the positive charge, towards the negative charge.
So alpha must be positively charged.
I said, OK, that sounds cool.
Maybe I can use this.
Maybe I can use this on this, on this question of how these things are spatially distributed.
So then he said, OK, let's just screen out those alpha particles.
And let's just take these alpha particles and let's shoot them.
So now comes the famous experiment, so you had stuff in here, radium making a beam.
And you collect it and you make it just alpha particles.
OK?
So now he's just got all alpha particles, and what he did is he also had a photographic plate, or this could also be a phosphorus screen, and you can watch where-- You know, if it's a photographic plate, you record events, and you can see it like a picture, a film.
And if it's a screen, then you watch it.
Either one was used.
But what he did is he put a very thin strip of gold in there.
0.7 microns of gold, so that's a 0.7 thickness is 0.7 microns.
Really, really thin, like a tissue paper of gold.
And he shot these alpha particles at it.
Now, why is this important?
Because-- gesundheit.
Because if this model of the atom was correct, then, you know, he'd isolated these positive particles that he's shooting at these atoms.
If that model of the atom is correct, then this is kind of like distributed all over.
Right?
And so it's kind of neutral almost anywhere you look.
It's kind of neutral.
And so you would expect that a positive charge-- you'd maybe feel a little bit, but it kind of feels mostly neutral because it's evenly distributed.
So it just kind of come out, but that's not what he saw.
That's not what he saw.
What he saw is that sometimes that would happen.
You'd get a little bit of deflection.
And other times, you'd get a signal out here, or there, or even right back at the radium.
How is that possible?
This did not work.
That model of the atom did not work.
And Rutherford himself said, it was almost as incredible as if you fired a 15-inch shell at a piece of tissue paper and it came back and hit you, because they just didn't expect that a positive particle could bounce backward off of an even distribution of charge.
And so this is a picture from your textbook, Avril, that I know you are actively reading on a daily basis.
So there's the alpha particles.
That's what I drew, that they would expect-- or that what I mentioned.
They would expect it to just kind of pass through.
This is what was found, where they kind of come back at very sharp angles.
That's what he actually observed, right?
And it led to the Rutherford atom.
And the Rutherford atom was that, look, the only way that this can happen, that you get these events, where a positive charge comes back like that, is if there's a very, very strong concentration of the positive charge in one small place.
And so he said-- let me see if I can read my date correctly-- 1912.
And so Rutherford, he said that the positive charge has to be in the middle, and then the electrons must be far away.
And that is also sometimes, it was called the planetary model.
And in fact, there was another scientist from Japan, Nangaioka, who, five years earlier, had predicted-- although he sort of predicted this, because he loved Saturn.
[LAUGHTER]
He really did.
So he said, you know, it seems that maybe we have a Saturn situation.
So he called it the Saturnian model.
But I want to mention it because it was part of the way there.
Right?
But in this planetary model, which came from Rutherford's experiments, you had a very different picture now of the atom.
You had a very different picture.
You had all the positive charge really concentrated in the middle.
Now, the thing is also that the atom, what they were starting to understand is that the atom isn't just-- like, there isn't just all this space here.
Right?
They knew the electrons weren't distributed evenly in here.
They knew they were sort of far away.
There is a lot of space.
There's a lot of space, that the radius of the atom is 100,000 times bigger than the radius of the nucleus.
Right?
So just to put that in perspective, so here's a picture.
There would be the nucleus, the head of a pin.
And this would be the atom, a stadium.
OK?
If you took all this stuff, all the stuff in a human body-- that means the protons, all the stuff in the nuclei, the neutrons-- ooh, I'm getting there!
And the electrons, and you take 7 billion people, and you put that all into one volume, it is the volume of a sugar cube.
So you know, so things are pretty empty out there.
Things are pretty empty out there.
How empty are they?
But this is where you find true meaning.
This is deep!
How empty is stuff?
Look, if I weigh the universe, you know, the universe itself, we're going from the atom and the electron, all the way up to the universe.
The universe weighs something like, oh, 10 to 60-ish kilograms.
That's over roughly $30 billion lightyears.
OK, now, if we just do the math, so you weighed the universe.
You know how big it is, roughly.
Plus of give or minus-- plus or minus.
Then that means that 10 to the minus 20% of the whole universe is stuff.
And the rest is empty.
That's deep, because we find meaning in all of it, don't we?
That's deep.
Think about that.
That's not just the universe, but the atom.
It's the same.
You know, so much of everything is nothing.
OK, planetaria, but there was a problem with the planetary model.
There was a big problem with the planetary model, because classical ENM told us that if, you know, if a charge is accelerating, if a charge is accelerating, which it is, because it's ughhh!
We think it's going around.
We think they're going around here.
They are not!
Friday, we'll know more about that.
But we thought they were.
But if it's accelerating, you know, to keep the circle, then it's got to be radiating energy.
That's what classical ENM tells us.
An accelerating charge loses energy.
So if you go with that, then the electron-- let's see, the stability analysis that we get out of something like that-- hang on-- is that the atom would be stable, four-ish 10 to the minus 11 seconds, five times, roughly.
That's not giving us a lot of time.
All right?
And so we were getting so far.
We had this great model, but it didn't go with classical ENM.
So atom's stable for that with classical ENM, oh!
Because you know what's coming.
We're going quantum.
Not now, I'm just preparing you for later-- Did I hear an oh?
Thank you.
Friday.
OK, so Bohr comes along, and on Wednesday, we're really going to go into Bohr.
And we're going to talk about how Bohr's model, how Bohr's model of the atom allowed us to understand how light and matter interacts.
That's a big deal, right?
But we'll go into that on Wednesday.
For now, I just want to tell you what he did, which is he thought about this problem a lot.
He's like, this can't be.
We need some way out of this.
And he wrote this paper, where he said the following.
He said, let's go over here.
He said, OK, "In order to explain the results of experiments on scattering of alpha rays"-- we just did that, scattering of alpha rays-- "by matter, Professor Rutherford has given a theory of the structure of atoms." And then Bohr goes on.
And he says, "Great interest is to be attributed to this atom model." Now, that is a serious diss.
Great interest?
If somebody calls your model of great interest, you know you are in trouble.
He might as well have said back then, you know, this model is of great interest because it's totally wrong!
[LAUGHTER]
And if you want to know what's up, come over to me and I'll tell you.
And that's what Bohr helped with in his work.
And what he did is he wrote down some postulates.
He said, look, the Rutherford atom is correct, but the problem here is classical ENM theory.
Right?
You cannot apply classical ENM theory to the orbiting electron.
You can't, because that's what happened.
So something's wrong with literally nothing less than classical physics.
Right?
He said, Newtonian mechanics still works, right?
Newtonian mechanics works, but not ENM.
So he said, we can go classical on how things are moving around.
F equals MA stuff, but not ENM.
He also postulated that the energy of these electrons, the energy that these electrons sit at or have-- we'll get into this a lot-- he hypothesized that that's quantized.
Quantized.
Quantized.
We're saying that word.
It's a beautiful moment.
Quantized.
Quantized means that it could only have certain values, certain values, which means that if this electron were to be in one value and then change into another, that the transitions are quantized.
The transitions of an electron from one energy level of the atom to another can only have certain values.
And that is what we get from the Bohr model.
And boy, did it explain things!
That's going to be the subject of Wednesday, as I mentioned.
Right?
But this gets us to the Bohr model.
And here's Bohr.
We're now-- oh, I got to draw this.
We got all the positive charge in the middle, and then these electrons can only have certain radii, certain energies.
They cannot continuously roam around.
They can't.
They're quantized.
That's the Bohr model.
And this is closed in 1912.
This is closing in on the last and final model, which we'll get to this week, which is where quantum mechanics comes in.
OK, now, we got one more thing to talk about.
So this is where we're going.
We're building up the full understanding of the atom.
But there's one more thing that I've got to come back to, and that is that, look, we just said there are these positive charges in there and these negative charges out there.
But it turns out that atoms can have different masses and stay neutral.
All right?
So we're now in a position to talk about this thing that we've been calling the nucleus and the fact that it's not just protons.
We're in a position to talk about that.
And so, you know, Rutherford gave us this idea of the proton, the particle, the proton.
And it wasn't until 12 years later that Chadwick found the neutron.
What the neutron does is it adds mass to the atom.
See, the mass didn't work.
So again, you know, if you know what the mass of the proton is, then you can add it up, and you get the mass of the electron, although it's like 1,000th as much.
But it doesn't work.
There's something else in there that's adding mass.
But it's not adding charge, because these things are electrically neutral.
So those other particles are called neutrons, and what it does is it gives us ranges of masses for the atoms.
Right?
It gives us ranges.
So because, you know, hydrogen now.
If you think about it, 99.99% of all hydrogen is just that thing on the left, a proton and an electron.
But sometimes, it has a neutron.
So it weighs more.
It's still got neutral charge.
It's called deuterium.
And then you've got tritium, which is unstable.
You can make it.
And these are called isotopes, as many of you, I'm sure, already know.
These are called isotopes, and so the isotopes of an atom, the isotopes of an atom with the same atomic number, different number of neutrons, different number of neutrons.
So atoms have these three things in them.
They've got the neutrons, the protons, and the electrons.
We've been talking today about the protons and the electrons, but they got to have these other things in them, or the mass doesn't work.
And so if you look at something like carbon, you see the same thing.
And now I want to get into how these things are written, right?
Because here's carbon, and there are those electrons.
They're now put out there.
OK, we didn't draw circles, so maybe this is around Rutherford's time when Avril made this picture.
And there is the pluses, and there's other things in there.
Right?
And those are the neutrons.
And so what we do is we have a nomenclature for this, which is the standard.
And that is that if we write an element like this, this would be the element.
And we put something here and something here, this is the mass number.
OK?
So that's how many protons plus neutrons.
Neu-- ah!
Neutrons.
And this is called the atomic number.
Now, the atomic number, I've been referencing this thing, this atomic number.
I've said, Mendeleev and many others, they put things in order of atomic number, maybe mass sometimes.
Mendeleev did the thing where he did those things and properties, right?
And the atomic number was just a number, until later.
And once we discover another type of ray, x-rays, then this has real physical meaning, right?
But right now, it's still just a number.
It's a number of the element in the periodic table.
Right?
But that mass number tells me how many neutrons I have and what the isotope is.
So now I go back.
I can go back, and I can look at carbon.
Oh, by the way, carbon-- I wanted to write this down before I say that.
Carbon, when you look it up in the table, you have 12.011 AMU, or grams per mole.
That's what's in the table.
But now you know why, because it's just an average.
And in fact, the IUPAC, which is a rocking body of the International Union of Pure and Applied Chemistry, I'm sure you've seen them on the feeds.
They hold meetings and in Vienna on a nonstop night of intense meeting and partying.
And they said, hey, maybe in 2011, maybe we should actually do this with the periodic table, just to show that actually there really is a range of-- if I take carbon out of the ground here or carbon out of the ground there, maybe they have different AMUs, right?
These are averages, and there are ranges.
So they're actually talking about different ways of even putting this into periodic tables, right?
But this is it.
And this gets us back.
Oh, there's boron and nitrogen ranges, right?
But what we do now, at least, is we just look at the one number, because that's sort of averaged over, you know, basically anywhere you can dig this stuff up.
And the stable isotopes.
Stable isotopes.
And it gets me to my last slide, which just comes back to the beginning, as I promised.
Right?
Because now I can say not only how many gallium atoms are in this strip, but I can tell you, gallium has two stable isotopes.
By the way, it's got a bunch of unstable ones, but it's got two stable ones, gallium 69 and gallium 71.
And I can now ask you, how many gallium 69 atoms are in this strip?
How?
Because I can again look up in the periodic table and find that in a gallium strip, I know how many atoms I have.
But if I say, well, 69 times x plus 71 times 1 minus x, that's going to equal 69.723 AMU.
That's what's in the periodic table.
But I now know that that's simply an average over all the-- right?
So this would be the fraction of 69 GA, right?
Because that's how simple these isotope problems are, right?
It's simply averaging over the potentially stable isotopes.
And I leave you with one more great mystery.
As you are pondering this, think about this.
There are two elements, ah!
There are two elements for which there are no stable isotopes.
Technetium and promethium.
Nothing!
Technetium, we use all the time.
80% of all MRIs use technetium, but it's not stable.
We got to make it, right?
That's pretty deep too.
How did that happen?
Why did that happen?
For the answer, you'll have to stay tuned.
Have a good night, and see you guys on Wednesday.