Flash and JavaScript are required for this feature.
Download the video from Internet Archive.
Description: Discussion of polymers, radical polymerization, and condensation polymerization.
Instructor: Jeffrey C. Grossman
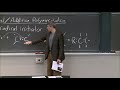
Lecture 32: Polymers I
we will be talking about a new topic today.
And that's polymers.
And we're going to start by talking about-- what I want to do is cover two ways that we humans make polymers.
And the first way has to do with something called the radical.
So that's where I want to start our story.
And what is a radical?
Well, a radical is a molecule that has one or more unpaired electrons.
So one or more unpaired electrons.
But as you can imagine, if I've got an unpaired electron, it's kind of like a broken bond.
It's kind of like a broken bond.
That's a radical.
And you could also imagine that a broken bond wants to not be broken.
So it's going to try to find something to bond to.
That's why we've got radicals all in us all the time.
Sometimes they're important, but sometimes they can cause a lot of harm.
And that's why if you eat blueberries, then you get these things called antioxidants.
And antioxidants, that's just one of many, many types of antioxidants.
What do they do?
They go and they give these radicals what they need, what they're looking for, an electron.
They give them an electron.
And they don't mind.
They don't become radical themselves.
They just simply stop the radical from being radical.
Now, why does this matter?
Well, we're going to see what that means for polymers.
But let's make a radical.
So imagine-- and I'm going to go Lewis on us, because I want to see the electrons.
I'm going to go Lewis.
So imagine I've got methane.
Well, methane looks like this, nice and happy.
Oh, the octet.
But now I introduced a chlorine atom.
So I've got now chlorine.
And chlorine is hungry.
Chlorine wants to fill its octet by itself.
And so what does it do?
It's like, well, I'm powerful.
I'm strong.
I'm just going to grab from methane.
It sees methane, and it's like, OK, you give me one of your electrons.
How about that is a deal?
And here we go.
And here we go.
And it takes it in the form of the hydrogen.
And so we got this like this, and like this.
Oh, the chlorine got really happy, but look at what happened to the methane.
It lost an electron and a hydrogen with it, and it has an unpaired electron.
That electron is not happy.
I mean, it just wants to bond to something.
That's all.
You can't blame it.
Don't we all want that?
The problem is that this really needs it badly.
And so this is called-- so what we did is we took chlorine, and we combined it with methane.
And we made what's called a methyl radical.
So this is called-- that's called a free radical.
Or in this case, it's methyl, because that's what the chemistry is.
It's a methyl radical.
As we'll see, you can make radicals out of lots of stuff.
Just take a hydrogen and an electron that have a free unpaired electron left, and it's a radical.
I could start with ethane and make an ethyl radical, C2H5 with a dot.
That dot is the unpaired electron.
And so on, and so on.
And so if you have free radicals and they come along, and there's some other molecules-- it's not going to be this one, but maybe there's something like an antioxidant in those blueberries.
It's going to come along.
I've got electrons I could give you.
Here, have one.
I see how needy you are.
I see how badly you want one.
You can take one of mine.
And I'll be fine.
I'll be fine.
Or maybe we'll form a bond with that.
Those are antioxidants.
But that's not the topic.
The topic is we're making this new material called a polymer.
So how does that work?
Well, to understand that, we've got to make a radical out of a special case.
We've got to make a radical out of a double-bonded molecule.
So now I'm going from methane to the double-bonded ethane.
Now, hang on.
Let me put this up here.
And in the case of a double bond, something very important happens.
Something very important happens.
And the reason is that I've got two bonds.
I've got two bonds, not one.
Well, that's what double bond-- that is what double bond means.
But I can now-- because I've got a double bond, I can do something very important.
I can do something very important.
When I turn that molecule into a radical, something special happens.
So what I'm going to do is I'm not going to call it chlorine.
I'm going to just call it R dot.
And because now we're making a polymer, and this is called-- well, it's got different names.
It's called radical, or sometimes you'll see it as addition polymerization.
And this is the first kind of polymer I want to talk about.
And so I'm going-- you saw how it worked with chlorine.
I'm just going to say, I've got something called R.
And it's called a radical initiator.
And I'm going to draw the dot there, just like I had the dot on chlorine.
This is going to be the radical initiator.
Radical initiator.
And I'm going to now bring this to my double-bonded molecule.
That's the key.
The key is in the double bond.
So watch what happens.
So first, here's what happens.
I've got R with its dot plus-- and again, I'm going to go Lewis.
I'm going to have all those electrons in the bonds spelled out for you with dots.
So R plus C. Two pairs of electrons in there.
Do you see that?
There's my double bond.
And here are the hydrogens.
I'm not going to draw them every time.
Those sticks have hydrogens on the ends.
But now watch what happens.
So now, this radical, like the chlorine, it sees this electron, and it says, aha, I can take you.
I can take you.
And I will be happy if I take this.
And this double-bonded molecule can't really stop it from happening.
So the radical now can bond to the carbon over here.
It's got these two.
Those are those two hydrogens that were there.
But now watch what happens.
Now I've got here, here, here.
And then I've got this.
And I've got this.
But now this is a radical, because this has an unpaired electron.
This has an unpaired electron.
And so often what we'll do is we'll write this.
We'll often just write it.
I'm just going to rewrite it, just because then it gets us in the mood for what's going to happen next as a single bond with the two hydrogens and the radical sitting there.
I just took this unpaired electron and put it out on the end there.
That's a radical again.
And I haven't broken the bond.
That's the key.
The double bond allowed a radical to come in, take an electron, make a new radical out of the whole thing, and remain stable.
That's what the double bond gave me.
So if I go to step two, what happens?
If I go to step two, well, now, I've got this R that's sitting here.
And I've got these here.
And I've got-- I'll put them in explicitly, and there.
Obviously, this is in a bucket of these C2H4 molecules.
They're all over the place.
And now this is going to see-- so we're going to do that, and this is going to see another one.
Let's put that one in here.
Here we go.
A double bond, nice and happy, floating along.
And this radical version of it sees it.
And it says, nope, I need that electron.
Thank you very much.
And so you get this.
Single bond, single bond.
And we'll just put it out here.
And so now, how many did I have?
2, 4, and here's another one.
And there it is.
And I've got another radical.
I've got another radical.
The thing stays radical until it finds another R dot.
So this just goes on and on and on.
This is a new radical.
New radical.
And each time it finds a double-bonded C2H4 molecule, it can take it, add it to the chain, add it to the chain.
Sometimes this is called chain polymerization, addition polymerization, radical polymerization.
You see it's all the same.
You see how it works, because I'm making a chain, and I keep on adding the same building block, the same molecule.
That's really important.
The same molecule, and then et cetera, et cetera.
Well, how long is et cetera?
Well, typical polymers go on for a long time.
We're talking a minimum of 100 times.
100, you might be able to draw that all the way down, maybe down to there.
But a lot of polymers go to 100,000 or a million chain links, a million.
That makes this class of materials extremely unique.
And we're going to talk about them in the next-- over the next-- well, during this week.
What I want to do is give you a couple of reasons why they're unique, and then we're going to go into my "Why This Matters." What we started with was a monomer.
In this case, we started with ethylene or ethene, and so here was our monomer.
And what we ended up with is a polymer.
And in this case, it's-- oh, boy-- I'll draw it straight like I did there.
OK, here we go.
Here we go.
This is the polymer.
But see, I don't want to write 100,000 carbon letters on my page.
You can you can't blame polymer chemists for that.
And so what we do is we have a notation, where if this was the monomer, one.
Poly, many.
Mer, C2H4.
Then what you do is you take the monomer and you write it in a special way to show that it's become a polymer.
And you put the monomer inside.
Oh, let's just write those hydrogens explicitly.
Why not?
There they are.
They were there, too.
But now what you do is you put parentheses.
And to show that it's a polymer, this bond comes out the edge.
That shows I had this monomer.
Now, notice, this is the same as a monomer, but a single bond.
Why?
Because that double bond, to keep on making it radical, I change them all into single bonds.
Each time I made the chain longer.
And then we put an n, because it could repeat n times.
And that's a polymer.
So the chain bonds are sticking out of the edges like that of the parentheses.
Polymer chemists aren't as-- the crystallographers, you don't want to upset.
Polymer chemists are fine.
So if you put brackets there, yeah, they're OK with it.
It's not so bad.
Parentheses, brackets.
The main thing is those bonds that are polymerizing, those bonds that are forming the chain have to come out of the parentheses, and then the little n there is how many times.
So a couple of things we can talk about.
So polymer, monomer.
Well, one thing, a couple of things.
So the molecular weight is going to be high.
It's going to be typically, and again, thousands of grams per mole, because it's a mole of the molecule.
This is a molecule.
This is a molecule.
It's just a very, very long chain molecule.
And so polymer people and bio people, they like using Daltons.
1 Dalton equals 1 gram per mole.
Why switch to Daltons?
I don't know!
It's just a unit thing.
So don't get confused.
Dalton is a gram per mole.
A kiloDalton is 1,000 grams per mole.
We can say like a Da.
Maybe they just like the symbol, Da.
Or kDa for kiloDaltons.
It's just grams per mole, thousands of grams.
But now one long chain, if I know the molecular weight in grams per mole, then I kind of also could know how long it is.
Back and forth, it's just a molecule.
It's just that this is a very, very big molecule.
And so sometimes you'll see it called a macromolecule.
Macro.
It's huge!
It's a long chain.
You just think about how the length is on the order of-- I'll spell it out-- microns.
Just think about it.
We've been talking about a lot of different materials in this class.
We've been talking about a lot of different solid materials.
Perfect crystals.
Crystals with defects.
Amorphous materials.
Molecular solids.
This is another class of solid, but you can really start to think about why this is so different.
Because if this thing is 100,000 or a million units long, that strand is a very long piece of spaghetti.
And that means that different things can happen.
And that's one of the reasons why polymers are so interesting and can be so useful.
And so if you think about taking it longer, this isn't even-- we're not even close to how long-- this is still just a little longer, but what I'm showing you is something important.
If I've got spaghetti that that's long, you better believe it can tangle up.
And so when you think about one of these strands or maybe a couple of them, they're going to usually, often, not always-- you can make crystals out of polymers, but a lot of times they're going to tangle, because they're just so long.
And the degree of the length, by the way, that's another thing, degree of polymerization.
Ah, why was my O so big there?
I don't know.
It was a moment.
I'm feeling it with the O. And that's the number of mer-- yeah, it's a polymer-- mer units.
And that's per polymer.
And this is average, because as you can imagine, it's very-- I'm going out to a million.
Did I hit a million or did I get 990,000?
Or did I get a million and 10?
You don't control the length exactly.
And so the degree of polymerization is the number that is the average.
It's the average.
It's the same as the molecular weight.
It's an average, because the strands have a distribution.
And we'll talk about that more on Wednesday.
But now I just want to get down some polymer insights.
So we got things like molecular weight, degree of polymerization.
Now, these are very long.
And so you can imagine that it's kind of like glasses when we did glasses.
These are really long chains.
You can imagine as a liquid that might be pretty viscous.
That might be pretty viscous.
You could also imagine that when you solidify it, it might be pretty disordered, or maybe crystalline.
It might have some similarities with glass.
Again, we'll talk about that more Wednesday.
But the one thing I want to highlight now is that what can happen because it's so long is that you can literally get what?
You can literally get like one polymer chain that finds itself wanting to form a crystal literally like in the middle of itself.
And then it's like, OK, I can stack and form a nice crystal, but now I can't again.
And now, oh, look, I think I can pack in nicely as a crystal.
And now I can't again.
And it just goes, I could-- I'm really enjoying this.
I could just keep going.
That's one polymer strand!
And it would keep going.
It literally would, because it's like 100,000 units long.
So you can imagine that polymers, just one strand of a polymer can be incredibly complicated, because it might have some regions that are crystalline and some that are amorphous.
And by the way, we know that the crystalline region will be very different.
This is going to have the lowest volume per mole.
So this will have-- maybe this will be the hardest.
Maybe it will be the most brittle.
So does it crystallize?
Does it not?
That's going to be something that's very important.
How much of it crystallizes?
A micron, by the way, the length is also important.
That's like visible light, wavelength.
So optical properties will depend on these things.
And so what you have is this new form of matter that we have in the last 50 years engineered and understood more and more how to control.
And so we're talking about this addition polymerization.
This list goes on and on and on now today.
So what is this list?
Well, these are all-- I want you to notice here-- monomer.
This is the polymer name.
This is the monomer.
Here's the polymer.
They should have put parentheses there.
We'll excuse that, because you can feel that those are going outside of the parentheses or brackets.
And then here's the use.
So what did we just do?
We just did polyethylene.
Polyethylene is right there.
That's the polymer polyethylene.
There is the monomer.
There is the polymer.
Now, notice something about every single one of these monomers.
There it is.
They've all got that double bond, that double-bonded carbon.
So when I put a radical initiator in a soup of those monomers, I can form polymer chains.
I can form polymer chains.
And those are the polymers you make.
And notice they all start-- these are the same unit repeating over and over and over again.
And notice the flexibility here.
So in polyethylene, which is one of the simplest cases and is the most common polymer, these are just a few uses.
If I just take one of those hydrogens off of one of the two carbons-- there's polyethylene up there-- if I just take one of the hydrogens off, and instead I put a benzene ring.
So now I've got this.
Oh, boy.
Did I draw that?
Yeah, it's OK.
Here's my hydrogens coming off of the benzene.
There you go.
There you go.
And there, and there, and oh, good.
I've drawn polystyrene, haven't I?
No, I have not!
Because you've got to come out!
That's a polymer.
Now it's a polymer.
I came out.
Well, that's pretty simple.
All I did is I just took the polyethylene, and I put a benzene ring there.
And I get Styrofoam if I put air through it as I make it processing.
Or I can make all sorts of other things out of it.
That's polystyrene.
That's polystyrene.
This is why polymers have become such an incredibly important and enormous part of our lives, because you can see if I just change the monomer, I just change one thing in one molecule, and then get a bucket of those.
I might completely change the solid that comes out of it.
But all sorts of things are going to change.
You can imagine that just the way the chain folds up on itself like that, maybe the way the chain comes and talks to another chain, or maybe a part of itself.
When I say talk and I'm talking about chemistry, I mean bond.
Is it Van der Waals?
The surface area is enormous here.
The surface area of one mol-- we did this, right?
We looked at boiling points.
As you get a little longer, a little longer, to C12.
This is C hundreds of thousands.
So the amount of bonding, London dispersion, is enormous.
That's one of the reasons these can be very strong.
But the tunability is also enormous, because I can just swap in a different group here, and polymerize that.
This is why this was so exciting when it was-- polymers were first made really in the '40s and '50s.
They really started coming into the market in the '70s en masse.
And now I want to tell you why this matters.
We're going to start with a commercial.
This is an ad on television by Pepsi in 1978.
And I thought this would be a nice way to start my "Why This Matters." It's only 30 seconds.
Uh-oh.
[VIDEO PLAYBACK]
Pepsi Cola's new 2 liter plastic bottle.
It's tough.
Really tough.
And besides being touch, Pepsi Cola's new 2 liter plastic bottle is 25% lighter than glass.
It's tough and light.
[END PLAYBACK]
Tough and light.
Now, apart from some other potential differences you may have noticed with today's times, that was the key.
That was one of the keys.
So they could make-- Pepsi and Coke, Coca-Cola, could make bottles that were lighter than glass, but not break.
That was a very big deal.
That was a very big deal.
And they really started-- in a lot of ways, they started the revolution of plastics and polymers in commercial products.
Now, if you fast forward to today, I just want to show you how bottles are made.
That's a 2 liter bottle.
Here is a 2 liter bottle making plant.
And I think it's always interesting to see how what you're buying is actually made.
So here's just a little short video.
[VIDEO PLAYBACK]
Harden almost instantly thanks to a built-in cooling system.
These preforms are now on their way to becoming single-serving juice bottles.
This is another plastic injection molding machine.
It uses the same method to make preforms for a different model, 1 and 1/2 to 2 liter bottles.
The preform's next stop is a machine called a reheat stretch blow molder.
In a matter of seconds, it heats each preform just enough to make the plastic malleable, then inserts a rod to stretch the preform lengthwise, while at the same time blowing in air at extremely high pressure.
This forces the preform into a bottle-shaped mold.
Cold water circulates within the mold to cool.
[END PLAYBACK]
So the reason I wanted to show you this is it just gives you a sense of the pace, the pace.
Now, the pace is connected to the material and the chemistry.
And it's things that you already know.
When we did stress strain curves, one of the whole regions of it is called plastic deformation.
These are plastics.
So one of the big advantages of these things is you can make like a small mold like you do first, and then that can be doled out to different processes.
And in a matter of less than a second with a little heat, a little pressure, it can be made into any bottle you want, and then the next one, and the next one.
Well, let's see.
We buy-- I'm going to write a couple of things here-- let's see.
We buy as humans 1 million of those bottles, plastic bottles-- anybody want to give me a unit?
Day.
It's per minute.
[GASPING]
We buy a million globally per minute.
And the thing is, if you're talking about plastic bottles, 91% of them are not recycled.
91% of them are not recycled.
And so my "Why This Matters," it's going to turn a little dark right now, because the thing is, if you compare with what nature does, these are nature's polymers.
And I will talk about them on Friday a little bit more.
And you can say, well, nature's had millions of years to work on this, sure.
Nature is the world's greatest polymer engineer.
We are all polymers.
We are all polymers of different types.
Amino acids, sugars, cellulose, keratin.
Nature does this in so many ways.
And of course, by definition, these are biodegradable.
Now, that's what nature makes.
Here's what we make.
Now, this is a beach.
That's a coastline.
And there's a lot of places where you can get some data.
I like Our World in Data.
You guys can take a look at that and many other resources.
Here's a beach.
Here is a guy.
It's hard to see, but he's actually in a boat.
That's a boat.
So that's all water.
And of course, there's a lot of information lately on things like how much of this stuff animals are eating.
And there's also a lot of pictures that are sometimes difficult to see, where animals get stuck in a piece of plastic, for example.
And that can lead to death, or deformity, as in this case.
And the thing is that we have to think very carefully about what we're doing.
And we have not done that with regard to polymers.
We have not done that at all.
And just to give you a couple more numbers, here's a chart.
This is how polymers are used today.
Packaging is 40%.
40% of plastic produced today is in packaging.
And the thing is-- sorry.
40% of the plastics are used only once, and then thrown away.
We all know that.
We drink a bottle of water out of plastic, or all the way back to the '70s, the Pepsi and the Coke, and we throw it away.
But the thing is that most of that, a lot of that, most of that winds up in the oceans.
And it takes about a half a thousand years, 500 years, to decompose.
So let's see.
A couple more numbers.
So first of all, only 7% of all plastic is recycled.
Now, Coca-Cola and Pepsi could use a lot more recycled plastic.
One of the main reasons they don't is the containers wouldn't be see through.
And we can't have that, right?
As consumers, we've got to see it.
It's got to be this beautiful, clear container.
But as I said, most of what's not recycled winds up in the oceans.
And let's see, in 2050-- here's one.
In 2050, the plastic in the ocean will equal the weight of all fish.
That includes whales and large sea animals, everyone.
Now, the thing is that-- let's see.
Many of these plastics, like polystyrene, there is an example, they decompose.
And so when they're large, large, there is a chance to collect them.
But the sunlight out in the ocean, they float up to the top or they get currents that mix them, and the sunlight, the UV energy from the sun, decomposes them.
Eventually they get smaller and smaller and smaller.
And eventually, they get really tiny.
Those are called microplastics.
You say, well, why is that bad?
Because it's toxic, for one thing.
Polystyrene is toxic.
The styrene molecule is toxic.
It's a carcinogen. It's a carcinogen.
People who eat seafood in some studies are estimated to be eating 11,000 pieces of microplastic a year.
That's what they're ingesting today.
So this is the problem.
We're making these single-use products, single-use, put it in recycling because I'm a good citizen, but 9 out of 10 times it doesn't matter, because it's not getting recycled.
Oh, and then it goes in the ocean where it stays for 500 years.
There's a plastic bottle.
There's fishing lines.
So this is the problem.
We should not be using things on the minute timescale that then take a half thousand years to decompose and are extremely toxic when they do.
This is clearly a problem.
Now, there are a lot of people working on this and thinking about this.
Not enough.
And I want to tell you about a book that I read when it came out a long time ago.
It's a wonderful book.
And I got reintroduced to it last week.
By David McKay, who unfortunately passed away recently, but he wrote this book called Sustainable Energy Without the Hot Air.
And I highly recommend you read this if you haven't.
It's free.
It's a free download.
WithoutHotAir.com.
Because he just goes through sort of simply the math.
And the reason I'm bringing this to your attention-- he doesn't really talk about plastics.
He talks about energy-- is I love this discussion in chapter 19.
Chapter 19 is titled "Every BIG helps." He says, if everyone does a little, we'll achieve only a little.
That is true.
We have this way of talking about these crises in little bits, because it makes us feel better, and it makes companies feel better.
You talk about this with the plastics in straws has gotten a lot of press.
And companies kind of do their part.
Although, Starbucks is kind of funny, because they replaced all plastic straws with paper straws encased in plastic.
That's actually more than the plastic straws to begin with.
Single-use plastic again.
But anyway, they're trying.
They walk away.
They say, we tried.
No!
Because that's doing a little.
The plastic in the ocean is 0.03% from straws.
There's so much more we have to do.
We can't think little about problems like this.
We can't.
We can't do that.
Now, there are some big projects.
This is one that's gotten some attention.
This is called Ocean Cleanup.
Now, they've gotten a lot of attention.
They've got these rigs out there that could collect some of this plastic in the ocean.
And it's at least thinking big.
There's a lot of bold claims that they're making.
A lot of bold claims, a lot of hype.
But at least it's an attempt to do something big.
One of the limitations here though is this technology can't go below a centimeter.
It can't go below that.
It's not going to collect below a centimeter.
But there are some studies that estimate that 90% of the plastic in the ocean already is below a centimeter, and 60%, 70% of that has already sunk.
Talk about the need to think about this in a big way.
This is a very big problem.
And I hope that more and more people get excited about trying to tackle it in big ways, not little ways.
That was my "Why This Matters," nice and happy.
Nice and happy, why?
Because we're going to solve these problems.
That's why.
We have to.
We have no choice.
I mentioned there were two ways to make plastics that I want to talk about.
I want to talk about the second way now.
So the first way is called radical polymerization, because I take one mer, and then I make it into a really long chain.
The second way-- and that right there is nylon, by the way.
Nylon can't be made that way.
Nylon can't be made that way.
And here's the reason.
Because in the second way of making polymers, we take two different types of mers.
And this way is called condensation polymerization.
It's a different way of forming very, very long chains.
So on Wednesday, we'll talk about what you can do with these chains, how you can modify the properties, kind like we do with glass.
We talked about the fundamentals of glass, and then we talked about engineering it.
On Wednesday, we'll talk about how to engineer the properties of these polymers.
But right now, I want to just focus on how to make them.
And so in condensation polymerization, the goal is also to make these super long strands, but you do it with two different starting molecules and no initiator.
So how does that work?
Well, I'll take a-- I'll get to nylon in a sec.
But first, let's just take something called the dicarboxylic acid.
And what I'm going to do is I'm going to leave a box inside.
And I'm going to say that could be something.
That could be something.
But I know that on that something, I've got this.
I've got a carbon, oxygen, and hydrogen, and on this side I've got the same thing.
OH.
So I've got the same thing on both sides.
That's a dicarboxylic acid.
It's carboxylic acid.
Now, on this side, I'm going to have another mer, and it's going to be different.
And this one, I'm also going to have a box.
And over here.
[SNEEZING]
Gesundheit.
I've got NH2.
And over here I've got NH2.
NH2.
Emphasizing the N is what's connected to the box there.
That's why I drew it that way.
And that's called an amine.
This is an amine.
And this is called a diamine.
So this is a diamine dicarboxylic acid.
And what happens is when those two molecules-- so the box can be anything.
The box can be whatever for now.
If it's got those ends to it, if it's got the carboxylic acid and amine-- so on the one hand, the molecule's got these groups on the end, and on the other hand, it's got these, then what happens when I put them in solution together?
Well, what happens is these two pieces react.
These two pieces react.
And so what you get is-- let's see.
You've got C. Here's my box there.
There's the O down there.
And let's see.
Now here's the carbon on this side.
Oh, boy.
I'm going to run out of room, and I don't want to.
So I'm going to move over here.
And recycle these boards so that I can draw it nice and big.
So now what happens?
So these are reacting.
And so now what do I have?
So I have C, double bond O. There's my carboxylic acid.
There's the box from the left-hand molecule, the one on the left there.
Here's another C, double bond O.
But look at what's happened.
I've formed a carbon-nitrogen link.
We'll talk about that in a second.
And then here's another box that came from the amine.
And this is an NH.
And then there'd be like another H there.
And there'd be another H-- yes, another OH here.
Yeah.
Yeah.
NH2.
So I got that back.
And what's happened?
What's happened is I've made water.
I've made water, plus I've connected these two molecules together.
So if I want to be complete about it, I've got to add a water molecule, because that's what had happened in the middle here.
The OH group saw the H on the NH2 group, and it said, hey, I want to make water.
Can I do that?
And then the carbon and the nitrogen were like, you know what?
Let me check it out, internally.
Let me check out my Lewis structure.
And it's like, yeah, you can take the OH and the H and make water, because then we could connect and form this bond here.
And that bond is called an amide link.
That's that kind.
And so I've formed a kind of bond between these two molecules that's a covalent bond.
And this polymer is a class of polymers called polyamide.
Because you can see now, OK, I've given off some water, condensation polarization, but I'm also now ready.
I've got my carboxylic acid, and my NH, my amine groups there ready to go again.
So if this side finds another one of these, it can attach and react.
And if this side finds another one of those, it can attach and react.
And it keeps going and going and going.
In much the same way, it's just now instead of a radical, instead of initiating it by tearing an electron out, I simply have brought together two types of molecules that want to, in giving up water, react and form a bond.
That's very powerful.
It's very powerful, because I can do-- I can now, like I said, this can have whatever inside.
So now it's a different way of thinking about it.
Before we had the mer.
And I could make the mer whatever I want.
Just keep a double bond somewhere, and I can make a polymer.
That's a lot of flexibility.
Now, instead of keeping a double bond, just keep these amine and carboxylic acid groups there, and I can put anything inside the boxes that I want.
Again, massive tunability.
And one of the funnest things to do is the nylon rope pull.
We couldn't do it.
Apparently, it's a little dangerous.
Nylon is when the box winds up having a carbon chain in it.
Six carbon atoms.
And when this has six carbon atoms and this has six carbon atoms, that's called nylon 6,6, which some of you may have seen.
So you get nylon 6,6, or sometimes it's just called 66.
It's six carbon atoms in the boxes.
You can imagine you can make 6,8, 4,6.
You can play around.
You can put lots of things in those boxes.
The nylon rope pull is such a cool thing.
I had to show you a video.
Here's what you've got.
You've got one molecule in solution on the top.
You can tell.
It's the slightly lighter liquid.
And the other one on the bottom.
The amines on one side, these carboxylic acid on the other.
This is nylon.
So the box is six carbon atoms.
Now, what's happening?
At the interface, this reaction is occurring.
So what I can do, and I'll play this.
Or what this person can do is put a little piece of glass stir in there and pull nylon out.
So watch how he does this.
So you get it started.
There is a piece of solid coming out of two liquids.
What's happening?
As he pulls it, more is reacting and forming more of these chains.
And you can just keep going and going and going.
What's so cool about this is you're literally creating this solid with functionality that's tunable out of these two liquid baths.
It's all happening at the interface where the two molecules, the amine and the carboxylic acid can see each other.
And there you can see it.
I wish you didn't have the text there, but look at that.
You're pulling a polymer right out of the interface between two liquids.
That's how nylon is made.
And again, this had an incredible impact on so many applications.
I thought I'd show you this one.
That's the first line in New York to buy stockings, nylon stockings.
And it's going on and on and on.
Of course, then there are a few other applications, too, like parachutes, and many, many, many more, because again, it's a whole other way of making polymers.
Now, I'll talk more about this on Wednesday.
But in your goody bag, you've got old school fun.
And we'll talk about this in the context of the things we're going to learn more about polymers and polymer engineering.
See you guys on Wednesday.