Flash and JavaScript are required for this feature.
Download the video from Internet Archive.
Description: Discussion of polymer properties and cross linking.
Instructor: Jeffrey C. Grossman
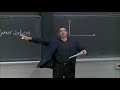
Lecture 33: Polymers II
Let's get started.
We're going to keep talking about polymers today.
Polymers-- that's also going to be the topic of the quiz.
And because of the exam and everything-- and so we're having our second lecture on polymers today.
The quiz tomorrow will be at the end of recitation so that you can have a full second recitation on this topic.
OK.
I mentioned this on Monday, right?
Monday we introduced polymers and I covered two different ways to make polymers, OK?
Let's write those down.
Let's write those down with chalk, which is somewhere.
Here we go.
On the one hand, we had what we called radical initiation.
So we use a radical initiator.
Remember this R dot?
And we talked about radicals, OK?
And how that can lead to this kind of chain reaction.
Right?
And so this is called chain polymerization.
Sometimes it's called addition, polymerization.
But it all involves a radical to start it and then a double bond.
Remember, we talked about that double bond.
The importance of the double bond.
Right.
So this would have some monomer.
This would have some monomer.
But it's got to have with a double bond.
That's not an equal sign, that's a double bond.
Now, the second way we talked about was called condensation polymerization.
So condensation polymerization these are two ways we covered that allow you to make these really, really long chains.
That's what a polymer is, right?
Polymer-- "mer" being from say hundreds to thousands and even millions.
And in condensation polymerization, you would have two different-- you could even have more.
--monomers.
And these two different monomers, well, let's write it explicitly.
They react, OK?
And they react because they've got these end groups that when they see each other, right, from one monomer to the next, they form a bond.
And in the case that we did on Monday, they formed an amide bond.
And they gave off water, right?
So water left.
So when you do condensation polymerization, you start with two different monomers.
They've got the end groups on them that when they see each other, they want to react.
You wind up with a polymer that weighs a little bit less than the two monomers, right?
Because you've given off water.
You could give off other things.
There's a lot of examples.
So in the reaction you give off maybe H2O, like we did.
Could be HCL.
Could be NH3.
There are a number of ways.
I don't want you to get the feeling that the only way you can do condensation polymerization is the nylon example, all right?
There's a lot of ways you can do this.
But one thing that is certainly true is that in forming that bond, you give something else up.
And that's why sometimes people like calling this addition.
Because in addition polymerization, right, you take a monomer and you just keep adding it.
And the sum is n times the monomer, right?
The sum of the weight.
Whereas in this case, it's a little bit less because you did a reaction.
OK.
So that's all kind of getting us back in the mood.
That's what we did on Monday.
Now today, I want to talk about the properties of polymers and how we control them.
How we can change them.
Different ways that we can change them.
I can't cover everything but I want to cover some key elements of engineering polymers.
So we're going to talk about the properties and a couple of specific things that we use to control them.
So let's start a list here in the middle.
And the first thing is something we already did talk about Monday, which is just the monomer itself, right?
So what is it that matters in a polymer?
What is it that dictates what a polymer will be like, right?
Well, one is the monomer.
So that's the unit-- well, OK.
We just said that you need two here.
So maybe there's monomers.
But that's the unit that you're repeating.
Clearly that's going to be really important, right?
What did you put there, right?
Is it C2H4?
Is it polyethylene or is it something else?
Did you add something to it?
The benzene ring, right?
And so that's going to be really important.
The other thing that we talked about-- and so we're going to create this list --is the molecular weight.
Now remember, molecular weight.
Oh we, like every little community, likes to come up with its own names.
We know grams per mole.
And then they say, oh that's a dalton.
And so now we have to say, OK, a dalton is a gram per mole.
Kilodalton would be 1,000 grams per mole.
But we also said that this gives us the degree of polymerization.
So that's really important, right?
Because how much to the degree of polarization-- I mean, if I know what my mer is and I know the molecular weight, the grams per mole of a strand, then I know how many mers went into it.
So I know the degree of polymerization.
That's just n, right?
It's some number.
But this is important and I'll put it over here so I can keep going down on that board.
When you have a synthesis of a polymer, doesn't matter which one of those you use, you're going out 100,000 units.
Yeah, I can't say well, every single one is going to be 100,000.
That is really, really hard to do.
So instead you get a distribution.
So when you say the molecular weight, what you mean is some average, right?
So this would be like maybe the molecular weight, maybe this is in daltons, maybe it's in kilodaltons.
And you say, aw, the molecular weight is 40 kilodaltons.
No, that's the average.
You might have ones that are way, way longer out here.
Maybe orders of magnitude longer.
This distribution can be orders of magnitude.
But it still can be you know, you can try to make them longer so you have a distribution that's more out here.
Or you make them shorter, right?
So this is something that is important and that can be engineered.
You can imagine if my chains are really, really long, then maybe it's a tougher material, right?
Because there's just more covalently bonded backbone to break, right?
You can imagine that that might be one thing.
Oh, but the thing that we gotta talk about is the chemistry which dictates the bonding.
OK, so the chemistry.
So the interactions.
And this takes us back to so many happy moments from this fall where we talked about intermolecular forces.
All of those apply to polymers.
They all apply.
What are the IMFs?
Well, if I gave you an example, like a question-- OK, maybe you could say, well, let's see-- OK, I've got polyethylene.
Remember, this how we write it?
And in this case, I've left off my Hs.
But the little sticks there means there's an H above and below each one.
I've got polystyrene.
So that's polyethylene.
Here's polystyrene.
Remember, this one has a benzene ring.
Oh, that's a terrible drawing of a benzene ring.
But it's what it's supposed to be.
They have to come out of the parentheses.
If it doesn't come out of the parentheses is not a polymer because you're not indicating repetition, right?
OK, that's supposed to be benzene.
And that'll be polystyrene.
And then how about another one?
I'll put it here.
How about polyvinyl alcohol?
So now watch this.
I'm going brackets because remember, it's OK.
And so here OH, right?
And each one of these would have some number of repeats.
That's polyvinyl alcohol.
I can say well, just look at those monomers.
Just look at the mers, right?
Remember, the monomers if these were made with chain polarization, the monomers would have a double bond, right?
Remember that.
But so this is the repeat unit in the polymer.
That's what goes into the brackets or the parentheses.
I can look at it and say, which one of these is going to be the strongest mechanically?
And you know from your IMF days.
You know which one it is because only one of them can form a stronger bond than London.
They're all going to have London.
Everyone has London.
But this one has hydrogen as well.
That's got hydrogen bonds.
So the polyvinyl alcohol will be stronger, right?
That will be the stronger one.
And you know that bond is going to be in there.
It's going to bond to other hydrogens from other chains or maybe from itself.
Because remember this is what it looks like.
But even much, much, much, much, much more.
These are macromolecules.
These are macromolecules.
OK.
Yes.
But now I wanted to put these here so I had them.
So I'm going to take one of these.
Which one is it?
It's polystyrene.
It's this one with the very poorly drawn benzene ring. .
And I didn't change anything about it but look at what it can do.
That's polystyrene in three different forms.
They're all cups but you know these cups well, right?
One's Styrofoam.
One, I don't know what you call that.
But they're all plastic cups but they have very different properties.
They have the exact same polymer.
Polystyrene.
How do we do that?
That's not the IMFs.
It doesn't even have to have anything to do with a molecular weight.
It could.
No, it's something else.
And that is the density.
Oh and so now we get to talk about density and crystallinity.
And I just saved three letters.
Makes me very happy.
Now crystallinity density, memories.
Good memories.
Very good memories are happening right now from when we talked about amorphous materials and the glass transition, right?
Because remember, I drew this on the board.
Let's see.
Let's get these to come down.
What that actually looks like-- each strand in there, remember, goes like this.
And then it's like, oh wait.
I can make a crystal.
And then it's like, nope.
I'm amorphous again.
And then it's like oh wait.
Hold on.
I can crystallize right here.
And then it's amorphous again and it's so fun.
And then you go like this and that.
And that's like one one hundredth of a strand.
And so you know the crystalline region-- what do I mean by "crystalline?" Well, the strand is stacking up in some ordered regular repeating way.
What do I mean by "amorphous?" Well, I mean spaghetti.
But a polymer, in its own strand, can crystallize to some extent or to more or less, right?
And that's something we can control.
We can engineer that.
And that's what leads to these differences because you know that in a crystalline region, it's going to have a lower volume, right?
It's going to take up less volume.
That's what we did before with glasses.
So let's put that on the board, right?
So you know that from out good old days that if I melt a material, well, it could form a crystal.
That's temperature.
And this would be volume per mole.
Right, this would be like the melting point.
It might form a crystal.
But with polymers, imagine I've got the spaghetti strands and now they're miles long.
It's really hard to line it all up.
And so a lot of it will not find that place.
And instead it will be a glass, which you know is another way of saying so these are now TGs, right?
TG.
And so you know now all about this, right?
And you know that cooling rate is one way to change the density.
To change the volume per mole, right?
So the density of the amorphous region is one parameter that could be glassy in different ways, like we did before.
And then how much of it you can make into a crystal is another parameter.
Both of which play into the overall density and crystallinity, right?
Both of those are extremely important and those are going to depend on all sorts of parameters related to the processing and the temperature and so forth.
Those are the things that can lead to changes like this from the same exact chemistry.
So let's go from polystyrene back to polyethylene.
So if you look at polyethylene-- so this is a list.
I showed I think a version of this on Monday.
It's hard to read.
Don't worry about it.
These are all monomers.
And this is the polymer.
They didn't put the brackets there but you can see-- oh maybe they did.
Yeah sort of.
You can see the line, right, coming out.
That's the repeat line.
And this is the monomer.
Notice the double bonds ready for chain polymerization.
And here are the properties and here are the uses.
Look at this-- LDPE and HDPE.
Now LDPE and HDPE.
So that's low density and high density of the same exact polymer.
So the interactions are the same.
But all we've done is we've changed the density and the difference between the properties is tremendous.
So when you go to the grocery store and they give you a plastic bag, which you'll wind up feeding to fishes in the ocean as you now know, it's very soft.
It's the exact same material that's in over 30% of all toys on the planet.
It's still polyethylene.
All we've done is mess with that.
We've messed with the density.
So how do you mess with the density?
How?
Well, it turns out with polymers there are different ways you can do this.
One we just talked about.
So one, let's do a little-- I'm going to do this.
So one would be let's say processing.
I'll call it processing.
That's right here.
How quickly did I melt it?
What were the conditions that I formed it in?
But see you can imagine changing its ability to crystallize our pack by just changing something else about the polymer itself.
And so another way to do that would be to change the physical structure of the chain.
Physical structure.
And there are two ways that you can do this.
Oh boy, let's see.
How am I going to continue this?
OK let's do this.
Bullet one.
One way is called branching.
And another is called tacticity.
So what are these?
So branching is exactly what it sounds like because the polymer I showed you here-- this is a mess and it's showing how it's a spaghetti chain.
But notice that it's just a line everywhere.
We would call this a straight line.
This is a straight line, it just kind of wanders around.
Yeah.
OK.
Because it's in contrast to-- if it's a straight line, then it's sort of locally straight.
But what if it did this?
And then it's like, well, which way do I go?
You can be the exact same chemistry but because maybe one of those radicals in the soup while this was being made came in and got to move a hydrogen around in just the right way.
It was able to start growing off of the side, right?
That's a branch.
So you can imagine that controlling this branching would be a really big deal in terms of the crystallinity right?
So now if you go to pasta.
It always seems to come back to pasta.
Maybe it's just me.
I don't think so though.
I think there's something deep about the pasta in here.
But see, this is a polymer.
But you can imagine now if I make spaghetti and it looks like the left, it's all packed in.
This is actually an interesting idea for a new pasta brand, right?
And what if each spaghetti strand looked like that?
And now you take those and you try to make your-- it would be actually pretty cool but it wouldn't pack as well.
You can feel it with the pasta, right?
You can feel it.
It's going to take up more room in the bowl.
I mean, it's the exact same thing with polymers.
So if you want a polymer to be stronger, to have maybe more crystallinity or higher density, HDPE, then you don't want very much branching.
So you better dial that in.
When you synthesize it, you better think carefully about how you're making this to prevent those kinds of side chains from growing, OK?
Well, tacticity is another thing.
And that's not the same as branching.
Tacticity is remember-- ah!
Where did it go?
I had it up there.
There's the benzene ring.
Are they all on the same side or are they on alternating sides?
How did this thing go?
Did two go on the same side and then one and is it random?
I mean, you can imagine that if I have a way to break the symmetry of the chain like this, then you can imagine that if I break it a lot so I'm atactic, then that's going to be harder to line up as well.
That's going to make it harder for the spaghetti to kind of fall right next to each other.
But even if this spaghetti had little you can call these little, mini benzine branches.
It's not branching.
But you can call them little branches.
But if they're all on the same side, then you can imagine they can still stack really well.
So when you have side groups like this, this is another aspect of engineering the polymer that you got to think very carefully about.
Because if it's isotactic or syndiotactic, then it's going to be-- so that's the physical structure of the chain.
--then it's going to be able to pack more easily and it's going to be stronger, right?
if it's atactic and we're talking about like a hundred degrees.
Same polymer, same chemical unit make the molecular weights the same.
All you've changed is whether there's symmetry or not.
And you can change the melting point by a hundred degrees.
Right, of the same material.
So that's another way that we engineer the properties of polymers, OK?
Same polymer garbage bags to Tupperware.
Same chemistry.
Isotactic to atactic.
OK, now we get to something really fun, which is cross-links.
And this is number five, OK?
So we're talking about ways to control polymers.
Cross-linking is one of the single most important ways to engineer the properties of polymers.
And it basically is exactly what it says-- you're linking two different polymer chains together across.
Cross linking, OK?
Now, it turns out the one-- remember, I showed you the video of the nylon.
It's called the nylon rope pole.
If you want to see that again, Google it.
It's so cool.
You have two liquids with the two-- it's a condensation polymerization example.
Two liquids with two different monomers and you pull a solid out of the interface.
And that's this.
That's exactly this.
And those are the molecules, these are the monomers that were in the liquid.
And there is nylon 6.6.
Remember, it's because you have a chain there.
And this is what you get.
And it keeps repeating.
And there's your polymer.
The reason I'm saying that nylon actually cross-links is because it's something very important.
It's not just that these have different interactions with each other.
No.
It's something a little bit more than that.
So if I look at this and say, OK, here's a carbon and a nitrogen. I'm going to just try to draw a part of this.
Carbon, nitrogen, there's a hydrogen, and oh boy, here we go.
Here's an oxygen. OK.
And it goes on.
Oh, I'm not going to draw what's in there.
It's 6.6.
And then comes another.
OK, nitrogen, hydrogen, and then it goes up to a carbon, oxygen, and then ah, 6.6.
Or whatever it is, right?
And then it goes to a carbon and oxygen.
This should have been like that.
And this goes to nitrogen and so on and so on.
Oh remember, this is called the amide bond.
That's cool, right?
That's the bond that formed.
That's the amide bond.
That's the bond that formed this polyamide, right?
We did this on Monday.
But look at what happened.
So if I have another one of these that comes up beneath it-- and I'm not going to draw the whole thing.
I'm just going to draw that part that has the amide bond.
You can see that if I have an oxygen here with the carbon and let's do oh boy, nitrogen, and like that OK?
And so on, then if this lines up-- but it can line up.
And then over here you've got a hydrogen.
And so you can get this kind of really nice line up to form a hydrogen bond between these chains.
You can.
It can happen.
And that can add, as we know, that can add strength to the material.
So that's a cross link because it's a very specific linkage between these strands, right?
It's not just sort of an average over different types of bonding.
It's the specific link that's in the chain.
Well, it turns out that that just seemed to happen.
Because of the chemistry that was there it can happen.
But look, we can also make it happen.
We can engineer polymers and we do to have all sorts of cross-linking chemistry.
And it's really one of the most single important ways to control the properties of polymers, right?
So I want to talk about that a little bit.
And I'm going to do it with maybe the most well-used, at least, example of what a cross-linker does.
We're going to talk about rubber.
Now, rubber is a natural polymer.
You can get it out of trees, right?
I think maybe I have a picture.
Yeah, I do.
Oh, poor Goodyear.
But we'll talk about him in a minute.
There's rubber coming right out of a tree.
And when we see rubber in this class we think of isoprene.
And isoprene is this molecule, OK?
So here's isoprene.
There's a CH3 and then there's a double bond to a CH2 and another double bond to another CH2.
Now, the polymer that is coming from this rubber tree is what?
It's polyisoprene, OK?
So I've got two double bonds.
We're going to talk about that in a second.
But I only need one to polymerize this.
I only need one, right?
And so if I think about this, I can make a polymer out of it and the polymer would look like this.
This would be polyisoprene, OK?
So H, I'll draw the Hs in just so we keep track.
Here's another C. And this has my-- oh boy.
I said I'd draw them and now I have to.
Once you commit, all right, there it is.
H, right?
And then you've got the double bond in there.
And an H there.
And then you've got this CH2 unit there.
Oh, and that is polyisoprene.
It's not-- it looks complicated.
It's got a CH3 unit and some CH2 units.
But here's the key-- it's got left over, a double bond.
All right, that's the key.
Now, you see, that double bond, I can do more chemistry with.
I can do cross-link chemistry with.
So I started with two and I'm left with one because I needed one to make the 100,000 long chains of these things, right so this is isoprene.
And this is polyisoprene.
Yeah, but the thing is that polyisoprene is not very useful.
The Mayans used it.
People tried to use polyisoprene directly from nature for centuries.
The Mayans would because it's soft, you know?
It's like a mold.
It's this really cool mold.
And probably, at some point, somebody stepped in it.
And they're like, look, my footprint.
And it stayed.
And so then, what they did is they made boots out of it.
And so they would put their whole foot inside of bucket of isoprene.
and then it would sort of mold really well, right?
And they'd make shoes that way.
The problem is people tried to do this much later too.
The problem with making a boot out of natural rubber is that the natural rubber doesn't hold together very well.
So like seriously, on a hot day, the boot melts, which is not what you want.
People made clothing out of it too.
Jackets were made with polyisoprene inside of two fibers.
But then it would melt and get like sticky, like, on a summer day or if it rained out.
So you had some issues with rubber.
But people really wanted to make something out of this stuff.
It seemed really neat.
It could kind of be strong but then very moldable.
And so along comes Charles Goodyear.
And he accidentally left rubber on his stove with sulfur.
And literally, the way the story goes is his house started smelling like burnt rubber, which was probably the first time ever that smell was made.
And what he found was that this rubber was completely different when it was left on the stove with sulfur.
It all comes back to the double bond.
It all comes back to the double bond.
Why?
Because now I've got a place where I can add a covalent link.
And that's exactly what sulfur does, all right?
So if I take this-- I'll try to draw it again.
I'm not going to draw the Hs this time.
So I've got this and here's that special double bond, right?
And then I've got this and this.
OK, that's natural rubber.
But now see, with a double bond, I can-- remember, what are these?
Well, these are four electrons?
But if sulfur comes along, I might be able to take two of them and move them like this.
If sulfur comes and offers something that the carbon wants.
Hey, come and bond with me.
It'll be more fun.
Maybe we'll go to a lower energy state, right?
And then the carbon's like, you know what?
I get that.
That sounds pretty cool.
I'm going to give an electron from this double bond.
I get to stay connected here with a single bond, right?
And then I can be here and here and the sulfur can provide that other electron and form a nice single bond, right?
Because there's that double bond, you can do the chemistry to create covalent strong bonds to sulfur.
And then you get like, another sulfur and another sulfur and so on and these can be maybe 2, 3, 4 sulfur units long, depending on how you cook it.
And then this will come.
And this comes down and finds another one, finds another strand.
And it finds the double bond that's in another polyisoprene strand.
That's called cross-- that's well, accidental.
He was a tinkerer.
And he played with lots of things.
And his greatest discovery was completely by accident.
And he never made any money off of it.
Goodyear Tire, this is Charles Goodyear.
Goodyear tires, this is the company.
It was called the Goodyear Vulcanite Company.
Goodyear called it vulcanization.
And the reason is Vulcan is the Roman god for fire.
And he accidentally left it on the fire.
And so that's called vulcanization because of that.
So this used to be the Goodyear Vulcanite Company.
It's now Goodyear Tires, right?
And what happened?
What happened is this low sulfur bonds that came about accidentally, the covalent bonds, held that material together.
And it gave it a controllable flexibility and hardness right?
That's what they were after.
That's what they were after-- flexibility and hardness.
And so what is actually happening in this?
Well, what's happening is you're adding these covalent bonds between these very, very long strands, right?
And so you can imagine that if you pull on that material now, well, the long strands can detangle.
So in the rubber tree, these long, long, long, long strands, they're all wrapped up.
It's a spaghetti bowl.
And I start pulling.
And they don't break but they start to kind of slide and detangle, right?
And eventually, you just break it all apart.
But now, I've got a lock on it because each little piece of spaghetti is tied to another one somewhere.
So I can untangle them only to a point.
And then these linkages kick in.
And they're like, no I'm sorry.
You can't stretch anymore, right?
That's what the crossing does, it gives it that strength.
And so you can imagine, if I had more and more cross-linking, I might have a stronger material, right?
The stress strain curve could go like that.
Not much cross-linking.
A lot of cross-linking, right?
Because more and more of it is going to be dictated by these linkages here between the strands.
And then there's something really cool that can happen with cross-linking because this is an example of a covalent bond.
This is covalent.
But cross-linking can be-- let's see, it can be covalent, it can be hydrogen.
We already saw that there's sort of natural cross-linking in nylon because of that hydrogen bond.
You can introduce hydrogen bonds as well.
You can be purposeful about it.
By the way, you can have ionic-- you can put any kind of bonding that you can dial in, and there are different ways to do that, can become a cross-link opportunity, right?
So you can just imagine how much this opened up polymer design.
How much this cross-linking opens up polymer design.
Now, there's a downside as well.
But first, let's say positive.
Let's go to your goody bag.
This is the chemistry inside your goody bag, right?
Now, this is a very purposeful.
Right, these are the Borax cross-linkers you've got that in your bag and you add it in.
And what I want you to do is get an actual feeling for the cross-linkers, right?
And so you can add more and you'll feel the material change because you're linking more and more of it.
But see, in this case, it's a hydrogen bond.
You see that?
See, those are the Borax cross-linkers.
Those groups keep their OHs and then those OHs, there's the polyvinyl alcohol.
I drew that on the board somewhere before and those OHs can now bond to the hydrogen or the OH and the PBA and that can form a cross-link.
But there's something different about this one and it's so cool.
And that's why slime is so cool and Silly Putty, right?
Because now there is a timescale involved.
There's a timescale involved.
So now you can pull it very slowly and the hydrogen bonds that are cross-linking haven't have time to change, to move, to reform.
Yeah, they break and then they reform, right?
But if you pull it really fast, those hydrogen bonds don't have time, right?
And I'll show you a picture in a second.
And so what can happen now is the mechanical strength of this material, the brittleness of it.
The properties of it can literally just depend on how quickly you pull it.
Because you are up against the speed of hydrogen bond forming and reforming.
And that is incredibly cool.
You are literally feeling the formation, the destruction and formation of hydrogen bonds.
But there's something else that's going on in this that's also so cool.
This material, slime, that polymer is below its glass transition temperature.
Sorry, above.
I meant above.
What does that mean?
It means that without the cross-linkers, that polymer is a viscous liquid.
We drew it somewhere.
I erased it?
I erased it.
If you're below the ground the glass transition temperature, you're basically a solid, like a brittle solid.
Well, like I drew, polymers are amorphous, maybe with some crystalinity but a lot of it's going to be amorphous.
Maybe all of it.
And so it's going to have a glass transition temperature and if you're above that, it's this viscous liquid.
It's this like super cooled viscous liquid.
But what I've done with the cross-linkers is I've said hold on.
You can't go away.
So I'm literally making a solid out of liquid.
We say yeah, just put like, water in the freezer.
No, that's not-- the phase of that polymer is liquid.
It is a viscous liquid.
But it is not allowed to flow or if it does, it maybe can change its shape a little.
Bit it can't just flow all over the place because of those cross-links, right?
And that's an elastomer.
And if you do it just right, you get a viscal material.
And what do I mean by that?
Well, all these things have to line up, right?
The thing has to want to be a liquid so it's got to have the right glass transition temperature.
But the cross-linkers are sort of holding it in place.
And the cross-linker chemistry has to be the right strength.
It can't be too strong, can't be too weak, right?
And again, by accident, you might make cross-link silicone.
Oh there's the goody bag, which is Silly Putty.
And that's why I wanted to give that to you because this hits all the right combinations.
In this case, the backbone is made out of silicon, right?
Not carbon.
But it's the same thing.
It's the cross-linking that does this.
So there's an example of Silly Putty, which you all have the goody bag.
And if you stretch it slowly, those hydrogen bonds-- the strands can kind of pull away.
And those hydrogen bonds have time to slowly form and reform.
That's why it kind of feels the same.
Nice, elastic right?
Depending on the material, on the backbone, it might go back as well.
In Silly Putty, it kind of just keeps on-- those strands just keep on sliding and the hydrogen bonds keep reforming.
But if instead I pull it fast, the hydrogen bonds don't have time to react.
That's why, if you pull it really fast-- like OK, don't do this.
Somebody shot a bullet through it.
It breaks like glass.
And you can feel it in your hands, just pull really fast.
The hydrogen bonds don't have time to reform, right?
And so what you're left with is you're just breaking apart this material.
Now, how elastic it feels, right, how viscous it feels.
All these things, these are going to be determined by the glass transition temperature of the temperature of the room, right?
And so that's going to depend on whether you actually have a very vicious liquid.
Or maybe you've got a solid, then it's going to be more brittle.
All of these things are now at your disposal to tune the properties of the polymer, right?
They're all at your disposal.
And it's incredible to think about, for me at least, holding together a liquid in place like that.
And that's leads to viscoelasticity.
Viscoelasticity, right?
That's just viscous liquid in elastic, right?
OK.
Now, there's a couple more things that we have to talk about.
And on Friday.
What I want to do Friday is I want to come back a little bit to some of the ways that we're trying to maybe engineer polymers to be more like nature, right?
We were talking about Monday, if you want to try to solve the problems, try to do something big.
And I gave an example of an ocean cleanup project.
But the ocean cleanup project wouldn't it matter if we keep dumping more and more plastic in the oceans that doesn't degrade.
All right, so we have to think very carefully about what kinds of plastics we can make.
And the problem with cross-linking-- this gets to the negative side, right?
And this is a way of categorizing these things that I've been talking about is if I cross-link it a lot, we call that a thermoset.
We call that a thermostat.
Why?
Because when you heat it up, "thermo," it's set.
So you mold it and then you've got your little cross-linker.
You know, you pour your sulfur in and then it's got a very high number of cross-links.
And you can see it there, right?
And it gives you very strong material.
But you can't break it back apart.
So it's very hard to recycle.
It's almost impossible.
If you try to heat these back up, they just burn usually.
And if you melt them, they're not usually that useful because they've got all this other chemistry and it completely messes with the polymer.
On the other hand, a thermoplastic has this kind of plastic deformation.
Think maybe like a garbage bag.
So it can really stretch and stretch and stretch.
But it doesn't have the cross-linkers.
That's why you can do that.
And so this is actually much easier, heat it up, melt it, and you can reuse it.
So that's a recyclable polymer.
But the issue there is it's very difficult to get all of the necessary mechanical properties from these thermoplastics.
And so elastomers, which are maybe lightly cross-linked polymers that are above the glass transition temperature often, those are somewhere in between.
But most of the time, even if you cross-link lightly, it's very difficult to recycle.
And that's a really good problem because maybe we can come up with cross-link chemistries that are reversible, right?
They can give us an opportunity to have the best of both worlds.
It's elastic when we want.
It's deforms when we want and it's solid when we want.
Oh and by the way, you can degrade all those cross-links and reuse it.
That's like one of the dreams in making environmentally friendly plastic.
See you guys on Friday.