Flash and JavaScript are required for this feature.
Download the video from Internet Archive.
Description: Prof. Short introduces a new invention which uses radiation-induced color changes in binary salts to function as a real-time dosimeter for proton cancer therapy, using physics introduced in this course. Sources of background and human-made radiation, levels of public exposure, and routes of exposure are introduced, and exposures in the form of cosmic rays, terrestrial sources, medical procedures, building materials, food, radon gas, and other sources are quantified to give intuition for how much radiation is naturally present around us.
Instructor: Michael Short
Note: To report potential content errors, please use this form.
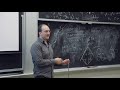
Lecture 31: Frontiers in Nu...
ANNOUNCER: The following content is provided under a Creative Commons license. Your support will help MIT OpenCourseWare continue to offer high quality educational resources for free. To make a donation or to view additional materials from hundreds of MIT courses, visit MIT OpenCourseWare at ocw.mit.edu.
MICHAEL SHORT: Hey guys. So quick announcement, we're not doing nuclear activation analysis today, because the valve that shoots the rabbits into the reactor broke and needs to be repaired. So we'll likely just do this next Friday. And instead, we'll have the whole recitation for exam review, like I think we'd originally, originally planned.
I also want to say thanks to whoever said, please write lecture notes for this class. It's something I think needs to be done. And I just now biked back from meeting with a publisher, or a potential publisher, to actually get this done. Because as I think as you guys have seen, there's no one reading that does this course justice. There are some that are too easy, there are some that are too hard.
And there are giant pieces missing, like most of what we're going to go into today-- sources of background radiation. And where do cosmic rays come from, and what are they? So thanks to whoever said that, because it spurred me off on a let's make this into a book kind of thing.
Want to quickly review what we did last time. We went over all the different units of dose and radiation exposure, from the roentgen-- which is pretty much just valid for those measurements in air. And I realized that yesterday I brought in the civil defense dosimeter and passed it to one person, and we didn't continue bringing it. So I'll bring it to recitation. It's not in my bag.
There is the unit of the gray, which is just joules per kilogram. Which you can calculate from stopping power or exponential attenuation equations. This is where you start, because it's how you get from the world of physics to the world of biology. Into units of increased risk, or sieverts, which is just gray times a bunch of quality factors, which are either tabulated, or I'd like to see somewhat better calculated. They are calculated sometimes, but by empirical relations, because that's usually good enough. Biomeasurements tend to be pretty sloppy, and I'm not that upset that these are empirical relations.
I'm going to skip way ahead past the detector stuff. We didn't quite finish up the IF2D idea. I think this is where we ended last time, where we were talking about how do you detect dose during cancer treatment. And I was outlining one proposed way that we're thinking of. Using this F-center based dosimeter, which changes color when it gets irradiated, you can implant it in the tumor. And as it moves from things like breathing or swallowing, you could feedback to the proton beam, and only irradiate when it's in range. Or play nuclear operation, and try not to hit the sides. However, whatever you want to do.
And there's multiple implantation options for this. We've thought about things like implanting a fiber optic cable into the tumor, and then having a port on the side of you that could do some in body spectrometry, which would be pretty cool. Could also put it all in a chip. You could have the emitter-- either a broadband or single color LED-- the F-center, and the spectrometer all in a chip that's implanted. And with radio frequency power transfer, so you don't need to put a fiber optic port and plug it into the side of you. Or however it goes.
And what we're doing next in this development is nailing down what color change is given by what dose-- so the physics. Develop an on-chip version. Find a bio-compatible casing. We've done the IP part, which is pretty cool. As you can see with patent office at least has taken in the application. But it's neat that you need to know pretty much everything you learned at MIT to pull off a project like this. From the nuclear physics stuff, to the material science, to the 22.071 electronics, to the medical stuff for biology, to the financial stuff for econ. In order to pull off an actual nuclear start up project like this, you need everything you learn here. Which is kind of a neat case study.
But now let's get back into what does a sievert really mean in terms of increased risk? Usually means the increased risk of some sort of long-term biological effect, whether it's cancer or some other genetic effects-- let's say mutations-- anything that would take a while to manifest, and would manifest by slow but steady cell division. And if you notice the difference between adults and whole population, let's see-- yeah, sorry. You can see right here that these-- not very much dose. Let's say in the realm of 10 to the minus 2 sieverts can give you some increased risk for cancer or some other effect. So we're talking on the realm of 40 millisieverts or so would give you some additional cancer risk. That's not a lot of dose. That's up to about the limit of the occupational dose that you're allowed.
So when we talk about how much is too much, I've taken some excerpts from this Committee on Radiation Protection document. This is from Turner, but the entire document, as I mentioned, is up on the learning module site. So you guys can see the actual verbiage where this is defined. So your lifetime dose should never exceed in tens of millisieverts the value of age in years. Which means in some years if you get a little bit less dose, you can get a little bit more dose and still be considered safe or not have any appreciable increased risk. And while you're working, you should never get more than about 50 millisieverts in a given year.
How was it for radiation workers? So for you guys, what dose are you allowed per year working at the reactor?
AUDIENCE: About five rem per year.
MICHAEL SHORT: Five rem per year, which is 50 millisieverts. Awesome, so it's--
AUDIENCE: [INAUDIBLE] to your eyes [INAUDIBLE].
MICHAEL SHORT: Oh sure, yeah. To jump back to that, if you're saying that there's actually tabulated differences for different organs, that's where they come from. Let's say you can take less radiation to the same organ and get the same dose in sieverts measured in equivalent risk. So I wouldn't be surprised if these are the organs that you're not allowed to irradiate as much.
AUDIENCE: [INAUDIBLE].
MICHAEL SHORT: I'm surprised the eye isn't here. Does it say retina anywhere? Oh, OK. Well that's just one table. It's not necessarily the complete answer.
Yeah, so 50 millisieverts isn't that much. Although if you think of it in the old xkcd units of banana equivalent dose, eating a banana gives you about 0.1 microsieverts. So you would have to eat 50,000 bananas in a year-- no, I'm sorry, 500,000 bananas in order to incur that.
Well yeah, I was talking to someone at dinner last night about the banana burning experiment where we measured the activity in becquerels. And then we can calculate how much dose in sieverts it would take. And he said, how many bananas would it take to get some increased cancer risk? About double this. It would take about a million bananas to give you about 100 millisieverts.
And I said, you know what else it would cost? And he goes, 100 millisieverts. No shit. And I said, that's right. Yeah. Yeah, he totally didn't plan that, but it just worked out that way. Yeah.
And then how much is too much? Let's say for the general public for a background for excluding things like natural background and medical exposures, you're not supposed to get about more than one millisievert just walking about outside. And you don't tend to get that much more. Why are medical exposures not included, despite them being pretty radioactive procedures? Yeah?
AUDIENCE: Because they're very targeted.
MICHAEL SHORT: They are targeted, and so they could give a lot of dose to certain organs. But the amount of dose isn't necessarily why we don't count medical procedures. Anyone have any idea? Yeah.
AUDIENCE: Was it because usually you wear a lead vest if you're getting an X-ray?
MICHAEL SHORT: In some cases, like if you go to the dentist, you'll get a lead X-ray. But let's say you get a chest X-ray. Why don't we care that a chest X-ray is way more than you get? Because these things tend to save lives. So you're absolutely willing to get extra radiation exposure that may have a delayed effect if the immediate effect is to save your life. You had a question? Or no. OK. Yeah, so we don't count medical things because chances are you're doing them to improve or save your life. So what's a little bit of radiation compared to let's say finding the blood clot or the aneurysm or whatever it would take?
And then how much is enough?
AUDIENCE: Yeah, that's the table.
MICHAEL SHORT: This is the table that you're familiar with? Yeah. They actually talk about the lens of the eye. And that's a heftier dose. But also, the lens of the eye is not a very massive organ. So this would mean do not stick remaining eye in neutron beam, right? Or you've seen that sticker, do not stare into a laser with remaining eye. Yeah, the same goes for the neutron beam ports coming out of the reactor.
But the lens of the eye can take a fair bit more dose per unit mass than the whole body. The lens of the eye is not a particularly fast developing tissue. It can cloud up with an insane amount of radiation exposure. That would take a lot more than 150 millisieverts to do, though. And then things like 500 millisieverts for skin, hands, feet. Pretty much just groups of muscle, bone, and dead skin that not much is going on biologically. Blood's flowing through it, but that's about it.
And notice that the regulations do differ a little bit, but on the whole, they're fairly similar. Same for the eye, same for the feet, same for the year. Cumulative is a little different. This says 10 millisieverts times age. This allows you a little bit more. Whichever recommendations you follow, they're all pretty similar. And our knowledge of how much dose leads to how much risk hasn't changed a ton in the last decade or so. There's been all sorts of arguments for or against it.
Has anyone heard of this LNT or Linear No Threshold model of dose versus risk? This is something we'll talk a lot about on the last day of class. This is the theory that the amount of risk versus the amount of dose is linear. And no threshold means that every little bit of dose gives you additional risk. This is not supported very much by science. I'd say it's not supported by science.
The converse argument is also not supported by science. We just don't have the statistics at super low doses to say what happens. But the official recommendation is that there is a unit of dose that we define as nothing, and it's 0.01 millisieverts-- about 100 bananas-- per event, let's say-- yeah, where does it say-- yeah, per source or practice. So eating 100 bananas in one sitting is considered to give you zero additional risk according to the official guidelines.
So the guidelines put in place do not follow the linear no threshold model. But anyone that would claim that one or the other model is absolutely correct has either got a huge sample size of people that we don't know about, or is probably extrapolating beyond what the data will tell them. So you'll see this argument flaring up quite a bit. For the last day of class we'll have you read some arguments for and against the linear no threshold model that aren't just blogs on the internet, but they're actual published articles that have passed peer review. And it's really hard to tell exactly what's going on at low doses.
But meanwhile, let's focus on what you do get that we can measure. The actual contribution from background levels is about 50% radon. This is a natural decay product of radium. It's just everywhere. It's here right now. It's all through the atmosphere. This is why you want your basement to be rather well ventilated, because it's a heavy noble gas that accumulates down in unventilated basements. So you don't actually want your house to be sealed up super tight, because you can have radon accumulation. Especially if you happen to live near a granite deposit or on granite bedrock-- which everyone in New Hampshire does-- radon levels are a little higher.
There's actually a story about a guy that used to work at a nuclear plant somewhere in Pennsylvania. I don't know where, but he lived on top of a pretty good granite deposit that was a few parts per million radium more. So the radon levels in his house or in his basement were much, much higher than would normally be allowed for background. And this guy used to set off the radiation alarms coming into work. Then he would breathe the nice clean radiation free air in the plant and go out without setting off the alarm. Eventually something had to be done. I don't actually know what was done, but if any of you can find that original story, that would be pretty cool.
Cosmic rays, another source that you can't shield from, is about another 10%. And we'll talk a lot about where these things come from. Terrestrial radiation, well we'll count that as stuff in the soil, stuff in the cinder block. Wood happens to be a fairly radioactive substance pound for pound, but it's not very dense compared to things like brick or-- well, banana ashes is probably about the same as wood.
Internal, coming from you. You'll have this on problems at number eight. Because you all now know your internal radioactivity thanks to going to environmental health and safety. Did anyone see anything disconcerting in your spectra, other than a tiny little potassium peak? Anyone? I'd say, ah, it's too bad. But that's great, especially for you guys that work at the reactor.
Medical X-rays. It's assumed that everyone gets a couple of these a year. You all go to the dentist and look for cavities. You break something, you may get an X-ray of your hand or foot. And this is let's say an average amount of medical X-rays.
Then a little bit of stuff leftover, consumer products. This isn't counting things like Fiestaware, those orange glazed plates and bowls that were painted with a uranium based neon orange paint. So we also don't tend to drink from Revigators anymore. Has anyone ever seen or heard of a Revigator?
AUDIENCE: Yeah, was this in the '50s or '60s?
MICHAEL SHORT: Or even earlier, yeah. So back in the '20s, people would put radium ore in these containers and pour water in it and say, natural radioactivity gets in. It cures croup or the Jimmy legs or astigmatoid rheumatism, or whatever other quack diseases there were in the '20s. You can still find them on eBay, and that's not accounted for in today's consumer products.
But this all accumulates the amount of dose that you tend to get on your own. You might get a couple of millisieverts a year of dose just from background, especially depending on where you are. And the big one-- whose spectrum I think you're all familiar with by now-- is that of radon. Because we saw most of these peaks in the bananas. Anyone have any idea why you would find so many radon decay products in bananas?
Given that radon's everywhere, we did notice elevated levels of specifically bismuth 214 and actinium I think 228 was the isotope we saw. Where would those come from? The what?
AUDIENCE: The soil.
MICHAEL SHORT: Absolutely, yeah. Whatever radium's coming out from the bedrock, that radon has to come up through the soil. If that happens to decay in the soil, it makes lead, bismuth, polonium, other heavy metals that are taken by the plant's tissues. In addition, radon daughter products can plate out on the leaves themselves. So this is one of those reasons that smoking is such a bad thing to do. Aside from the chemical effects, you have giant fields of high surface area tobacco that you then concentrate and dry up into these tiny little sticks. You have an enormous amount of leaf surface area and dry vegetation that has taken all these radon daughter products. So most the dose you get from smoking is lead, bismuth, polonium, actinium, radium. Alpha emitters.
As we saw last time, to remind you guys of the quality factor for alpha particles, it's as big as it gets. Anyone remember why that is? Why are alphas so damaging if they get into your tissues?
AUDIENCE: Because they're big.
MICHAEL SHORT: They are big, so they have high mass. And?
AUDIENCE: Short range.
MICHAEL SHORT: Short range coming from their high relative charge. They have quite high stopping power. And they will deposit a lot of energy very close to where they are emitted. So their range is very small. So that armor piercing bullet analogy comes to just an armor piercing bullet that explodes right out of the barrel of the gun. And they do quite a bit of radiation damage. We jump back to the right slide without inducing a seizure.
I think we've looked before at the radon decay chain. This is a simplified version, because there are different branches or different possibilities for decay, but some of them have extremely low percentages. So this one's simplified quite a bit. But it is whenever radon decays, it gives off a bunch of alpha and beta emitters that last anywhere from minutes or seconds to days and so. For every radon atom that you absorb, you end up getting four or five alphas and betas, depending on how long it stays into your system.
And then mapping out radon in the US. You have quite different amount of radon dose depending on where you are. And I wanted to skip ahead and overlay a couple of maps of the US. So this is a terrestrial-- oh, I'm sorry, that's the wrong one. Let's just look at this one, yeah.
Anyone notice any patterns here? Where do you tend to get the most radon? What sort of features would one live near when you get a lot of radon dose?
AUDIENCE: Mountains.
MICHAEL SHORT: Mountains, which tend to be made of?
AUDIENCE: Rocks.
MICHAEL SHORT: Rocks, which tend to contain a lot of radium. Especially granite and other such rocks. The Conway granite, named after up in Conway, New Hampshire, is about 52 parts per million uranium or radium. So it's a fairly toasty rock. You can actually tell there with your Geiger counter, if its count long enough, that there is a little bit of radioactive ore in that Conway granite. Not nearly enough to matter at all, and certainly not enough to stop you from making fancy kitchen countertops. But I wonder if folks would buy those if they knew that there was 52 parts per million of something with a half life of 9 billion years. I somehow think it would matter to people, but it really doesn't.
AUDIENCE: They put the radiation symbol on it [INAUDIBLE]
MICHAEL SHORT: Yes, engrave that. If they made the radiation symbol a little induction stove, that would be pretty slick. Yeah.
And then in terms of relative radon risk, how much actually matters? I like this graph. Despite being difficult to read, it actually shows how much the average indoor level compares to all the different things you could do. Like getting 2,000 chest X-rays per year versus 100 times the average indoor level. That's about what I heard that fellow in Pennsylvania had is like 100 or 80 times the normal radon levels from living underground on this giant granite deposit. It's like getting 2,000 chest X-rays per year, or something like smoking four packs a day.
I know some people that do this. They don't tend to be that afraid of the radiation that they're taking in from smoking. But yeah, it's pretty insane how much radiation you get. People are afraid to get one chest X-ray, which is not even on this map. One chest X-ray worth of radiation gives you much, much less than living for a year in a house, which we all tend to do. Then if you live in a brick or cinder block house, you actually get a fair bit more radiation, because these are fairly radioactive building materials. And I'll show you those activity levels in just a sec.
As far as exposure sources, again, to look at the relative amount of terrestrial gamma ray exposure, you can correlate that pretty well with not green regions on a topological map. So look at the really low levels down here, correlates with low lying vegetative areas around here. Colors are a little more extreme on my screen, but you can see this is all low lying right here. From Louisiana, Florida, up the east coast, until you get to the Appalachian Mountains and such. So pretty striking correlations there.
And it all comes from what we call the primordial nuclides. These are unstable nuclides that have such long half lives that they still exist, despite the universe being 15 billion years old, or whatever supernova formed our solar system being five plus billion years old. Things that we've already studied almost to death, like potassium-40. And you can see that's about 0.011% of all potassium is radioactive potassium-40. About 10% of which gives off gammas in-- what is it, 90/10? I forget the split. But can give off either betas and then a gamma, or positrons and then a gamma.
Then things like rubidium, in the same column as sodium, potassium, and cesium, so it behaves kind of like a salt-like element. There should be some for-- are there any for chlorine too? Some of the other important ones to note, oh, platinum. Does anyone has any platinum jewelry? Does anyone have any platinum jewelry? Good answer, yeah. I don't either. I teach at a college. Also, I don't like wearing jewelry.
But there'll be all sorts of these primordial nuclides that you can't really do anything about. They're just there. Note that the half lives are really, really long. And as you know now, the half life is going to be inversely proportional to the activity. Despite almost all indium-- look at that, 95% of the indium that you'll find emits betas. Doesn't stop people from using it as these awesome glass to metal seals for vacuum components. Because once in a while it might emit a beta ray, like a whole gasket might emit a beta once every millennium or so. But these half lives actually are measurable.
And that begs the question, are there elements with longer half lives that are just too long to measure? You think what does it mean to have a half life of 10 to the 15 years, given that the universe is on the order of 10 to the 10 years old? Is it possible that all nuclei will decay till the end of time?
I've seen some documentary such things and don't call this a science that say, oh 10 to the 40 years from now, the last protons decay, or the last whatever elements decay into all protons and neutrons. Don't know if that's true, but it does make me wonder, are some of the other so-called stable elements just have ridiculously long half lives? It's something to think about.
So let's take a look at the building materials, and see what's in the typical things around us right now. You can see how much granite, radioactive thorium, and potassium are in these building materials. And how many usually is measured in picocuries per gram. A picocurie is already less than a becquerel per gram. Because a curie is what, 3.7 times 10 to the 10 becquerels. And a pico is a 10 to the minus 12. So things on the order of a few picocuries per gram, a gram of that material might emit one disintegration per second. Not a lot of radiation.
But take a look at how much potassium there is in granite. Nanocuries per gram, that's getting into the integers worth of becquerels. If you look at wood, check that out. Anyone heard of potash before? It's one of the ways we get potassium. So if you take wood based things and you burn them in a fire, you drive off all the carbon in the water, you're left with these kind of whitened salt and pepper ashes. That's potash. That's the ashes left over in the pot after you burn stuff in a fire. They're quite potassium rich.
So I think what we'll do next year, instead of burning a bunch of food, is just burn a bunch of wood. We'll have a nice bonfire, collect the ashes. And we can see how much potassium there is in wood. Which pound for pound, if you see on this list, is the most radioactive building material there is. Just that wood happens to be pretty inexpensive, and consists mostly of water, lignin, and other carboniferous materials.
They don't have carbon-14 on this list, but let's take a look at some of the other ones. So sandstone cement has a pretty toasty signature to it. Gypsum drywall. What is it, 13 parts per million uranium in all the drywall you tend to find. Anyone scared yet? Because you shouldn't be. It's like I mentioned before, this is the slide I want to show to most folks in the general public. There is such a dose as nothing. And that's pretty much what you get from, let's say, a day's worth of being around these building materials. It's just about nothing. It's after the building materials. There we go.
Seawater is another great source of radioactivity. Enough so that people have actually proposed harvesting uranium from seawater. So the total amount of activity in the ocean, there's something like 11 exabecquerels of radiation contained in the uranium in the seawater. Which means you should be able to have a gigantic trawler flying out around in the ocean, and just floating through the seawater, picking up atoms of uranium here and there. Because technically, there's enough to power the world for like 2,000 years. The problem is the ocean is big. It doesn't stop people from actually working on it. So it's neat to think that there's a whole lot of carbon-14 and tritium and uranium in seawater. 300 picocuries per liter.
Anyone have any idea why there's so much more potassium activity than anything else in seawater? It's because it's salty, yeah. And potassium's just like sodium. So there'll be a fair bit of potassium in the seawater, 0.0117% of which is potassium-40. And so last year, people asked, what, uranium from seawater? How is that even possible?
So this is the part in the course where I'm going to pull out a lot more recent papers to show you some of the cooler innovations going on. So you could use this adsorbent. And adsorbent. Does anyone know what adsorption means, not absorption? Adsorption is when something sticks to the surface of something. Absorption with a B is when it actually gets incorporated into the bulk. So folks are thinking about making high surface area materials that can adsorb selectively atoms of uranium. So you send out this huge braided net, or a huge stack of adsorbing material with a D, and just go around in the ocean. Attach them to tankers or cargo ships, and just have them pull in product as they go from coast to coast.
And by actually changing around the chemistry and the geometry of this, you can enhance things specifically for uranium by about a factor of three. And this yellowcake right here next to an actual ruler-- that's 50 millimeters right there-- that was obtained directly from seawater. So you can actually pull yellowcake out of the ocean. Just not very much of it.
And the way these work is there are interesting compounds that selectively absorb uranium into their structure, not by direct chemical bonding, but by getting something close. For example, my wife tends to study metals bonding to proteins. And it's not necessarily always a full chemical bond like you might think, but the protein can kind of wrap around a metal ion, transport it places. Similar thing going here. I'm not going to pontificate anymore on it because the title has the word organic in it, and I am definitely not an organic chemist.
Don't want to tell you anything wrong, but I do want to tell you you can actually see this paper to find out what sort of compounds selectively grab onto uranium. And if there's a seawater floating through, and uranium happens to flow nearby, it can bond to it. You can then somehow squeeze out or burn off that adsorbent, and there you get uranium.
Now let's talk about what's in the body and interpreting the spectra that you got from EHS, your full body spectra. If you take a look at how much uranium there is in the body, wow, there's some. Every one of you is got about a becquerel of uranium in the body. But if you look at the relative amounts, rate it was at. The only things that really matter a ton, potassium-40, about 4.4 kilobecquerels per human. So each of you is giving off 4,400 potassium gammas per second. Most of them just flow right through all the other people.
And then carbon-14, about 3.7 kilobecquerels. Pretty interesting to know just how much radioactive stuff you have in your own body. You will need this table for homework number eight when I ask you a pretty fun question.
Then there's all the various medical procedures. The ones that aren't counted in your annual dose because they tend to save people. But now let's look at the dose in millirem So if you want to figure out what this is in sieverts, just divide by 100. So a regular old chest X-ray, 0.1-- let's see, how does it go? 100 rem in a sievert. Yeah, so about 0.1 millisieverts, or 1,000 banana equivalent dose. Not terrible.
Which is why if we go back to that chart of all those relative risks, if you look at the average indoor level, it's like getting-- well, I would have to guess maybe 40 chest X-rays per year based on this rather crude scale. And you're allowed to get around a millisievert or a few millisieverts of radiation per year. That sounds like it checks out mathematically.
Let's look at some of the other crazier ones. Dental bitewing. Yeah. So anyone ever bite down on something where you have to get an X-ray through the side of your mouth? Quite negligible amount of dose, yet they still put the lead apron on you. I'm guessing that's mostly for show, because that's very, very little dose. It's well beyond the 0.1 microsieverts of something that counts as nothing. But there's not a lot of dose going into these things. Let's see what really does give you a whole lot of dose.
10 millisieverts for a CT scan, or a whole body CT screening. That's a pretty hefty amount of dose. Right there with one procedure you may get more than your normal annual background dose. But if you're going in to look for stuff, chances are you need to find whatever you're looking for. So we don't count it. And it may give you a slightly higher chance of developing cancer much later down the line when that cell that gets mutated divides. But it's probably going to save your life in the next hour, or the next day. So definitely worth it.
Let's see, the worst-- what's the worst procedure we can find? Noncardiac embolization. I don't know enough biology words know exactly what that means.
AUDIENCE: I know what noncardiac means.
MICHAEL SHORT: Yeah, I think we all know what noncardiac means. Good. This isn't 7012.
Nonmedical procedures-- or more medical procedures, I'm sorry. Where's the techneitum scan? Yeah, notice how many of these things have technetium imaging where you'll inject technetium into a certain organ or a certain vascular or lymph or whatever system. Some of these things can give you a fair bit of dose. Like again, maybe a heart stress rest test can give you double or triple your normal background dose. This is why you have to declare to airports if you've just had a medical imaging procedure, because this is well more than enough to pick up on any sort of airport radiation monitor. So again, if you ever get a medical procedure with any sort of radiation, anything, do declare it, because it's quite measurable.
Then there's radiation from altitude. I may have mentioned already that the reason that pilots can't fly for a certain number of hours is not fatigue, but it's radiation exposure. When you start to look at how many microsieverts you get per hour on the ground, 0.03. Right about down at that-- hanging around for an hour is a negligible dose. You go up to international air travel, that goes up by a factor of little more than 100. And so you get a fair bit of radiation exposure.
If you take your annual allowable occupational limit of 50 millisieverts, divide by 3.7 microsieverts, you're getting close to 86-- what is it? How many hours in a year? Let's see. There's three times 10 of the seven seconds in a year. So divide that by 3,600. That's getting on the realm of 10,000. That's about the conversion factor. So you can't spend your life in the air, because you'd get too much radiation according to the occupational risks.
And so actually in addition to some interesting measurements that have been published in papers, we've actually had students go out and build radiation altimeters based on the MIT Geiger counter-- removing the speaker, of course, because you don't want to clicking Geiger counter on a plane. That'd be kind of a stressful situation.
Let me show you one example of these. Are also published from a paper, but we have pretty similar data from-- if you want, to go talk to Max Carlson, one of my graduate students who hooked up his Geiger counter to an alarm clock-- the case that had [INAUDIBLE] in it. I think this was a poor choice of case for a plane because it looks kind of like a time bomb. But luckily nobody found it, and he was able to get the data. But you can see just how much more data you get. And you can correlate the height that you're at with the dose-- in this case, microroentgens per hour or microrad depending on-- what is it-- ambiguous unit definition right there. But it's quite noticeable.
So for those of you who have built Geiger counters and have cell phones, and don't want to have a fake time bomb, you can actually hook in your Geiger counter to the microphone port of your cell phone. And with a few available radiation apps, you can actually monitor your dose in microsieverts. Assuming that it all comes from gamma rays, which is most of what a lot of cosmic rays will produce. So it's a pretty safe approximation that your dose in gray flying on the plane is also your dose in sieverts, because it's whole body, and its gammas. So I'd say try this at home, kids. This is one of those things I recommend you try out.
Speaking of these cosmic rays, where did they come from? Well, this is a question that's been under debate, and was more completely answered just a few years ago. They come from very high energy particles from somewhere. It had been argued, do they come mostly from solar flares, or did they come from elsewhere in the galaxy? Mostly we're talking about things like high energy protons or other charged particles.
We're also talking neutrinos. Anyone know about how many neutrinos are theorized to pass through you every second? Trillions. Yeah, something like that. But they basically don't interact with matter. As I showed you guys near the beginning, it takes a gigantic salt mine full of water and exploding photomultiplier tubes in order to catch two or three neutrinos a day if you're lucky. So let's say those don't really matter in terms of background dose. But when those high energy particles interact with the oxygen and nitrogen up here in the atmosphere, they produce a shower and cascade of additional ionization and high energy particles.
So it's been said that solar flares and such will accelerate charged particles from the plasma in the sun towards Earth. They're deflected somewhat by the magnetic field of the Earth, but they tend to enter right here at the-- what is it? At the poles. I'm sorry, that's the simple word I was looking for.
Until recently in 2001, they were looking specifically at the evidence for or against the idea that coronal mass ejections-- which means large ejections of mass from the outer layers, these sparcer layers of the sun-- was responsible for most charged particles and cosmic rays. Skipping the stuff that's not in bold, it appears to be that the CME bow shock scenario has been overvalued. So for a while, folks were saying most cosmic rays come from the sun-- that's our nearest star. By making really, really careful measurements of the energy and lifetime of these cosmic rays, this has actually been somewhat disproven that this is the major source of cosmic rays, which is pretty cool.
But let's talk about where they actually come from. Reactions that you can probably understand. So extremely high protons enter the atmosphere. They all start as high energy protons. And when protons are high enough energy-- and like I do probably in every class ever, I'm going to pull up Janis to show you something. They can undergo what's called spallation. It's the same principle that the Spallation Neutron Source at Oak Ridge National Lab works on is shoot in extremely high energy protons, out come neutrons.
So as usual, it didn't clone the screen right. So just bear with me for a sec. I'd like to, for probably the first time in this course, switch databases to the incident proton data. Is that actually working? OK, good. So we'll leave the incident neutron data, we'll go to the incident proton data. We'll stick with the same library.
Let's see how much they have. Not much, but enough to matter, because there's a lot of nitrogen-14 up in the air. Let's see what happens when protons hit nitrogen-14 all the way at high energy. So don't quite know what a negative cross-section refers to. But at high energies, this is definitely a possible event. And let's see, there's not a lot of cross-sections to look at here. Let's try oxygen-16. Not much. We'll stick to the slides then.
So when a high energy proton strikes a nucleus, it can eject neutrons. And those neutrons can then cause activation reactions, and then emit things like proton or tritium, leading to-- that's where your carbon-14 comes from. Comes from nitrogen-14. So in comes a high energy proton, releases a neutron. That neutron hits nitrogen-14, releases a proton, out comes carbon-14.
So this is why it's being constantly generated in the atmosphere. It's not like there's a certain amount that was there at the beginning of the universe and decays, because its half life is only 5,700 years. So this is part of why radiocarbon dating works, because we have cosmic rays. It's kind of a neat connection to make. If there weren't cosmic rays, all of the carbon-14 would decay pretty quickly in the universe time scale. And we wouldn't have this form of radiocarbon dating.
And then same thing for tritium production in the atmosphere. This is where some of that tritium naturally comes from, is it makes carbon-12-- which is the normal form of carbon-- but out come tritium, which there is going to be some constant isotopic fraction of tritium in all the world's hydrogen. Some of it's being generated in real time.
And we do have these spallation sources on Earth. Like I mentioned, the Spallation Neutron Source has a gigantic synchrotron. We've seen these before, which just injects in this case protons, which circle around and around and around, accelerating until some of them are extracted and fire onto things like a liquid mercury target, some neutron rich liquid metal.
So you want something that's very neutron rich. You want something that's very dense. You want something that's fluid so you can cool it better. And you want something with high thermal conductivity. That's where the metal comes in. So a liquid metal you can keep cool really well. Because when you're firing lots of 800 MeV protons into it, you generate a tremendous amount of heat.
And this is what the actual thing looks like. You can get tours of this down in Tennessee at the Oak Ridge National Lab. Actually, I've driven up here before. I recognize this from the map. That's pretty cool.
So where the actual neutron science stuff happens, where all the scientists sit with their targets, there's quite a bit of stuff going on behind it. So there is a gigantic-- you can see that's a parking lot for scale. There's a gigantic linear accelerator shooting into the synchrotron ring, which then fires the protons here into the target into one of any number of end stations, which creates a not quite push button, but turn on-able pulsed neutron source, which is pretty slick. And again, parking lot for scale. Takes a lot of magnets to bend an 800 MeV proton.
That's what it actually looks like. Has anyone ever seen one of these synchrotrons? Like at Brookhaven or at Oak Ridge or at anywhere? They're quite interesting things. The closest one to us is the NSLS, or the National Synchrotron Light Source version two at Brookhaven National Lab. It's like a 2 and 1/2 hour drive down in Long Island. I don't know if they're doing tours yet, but it's about a kilometer around. And I was told they did bike races around it to see who could beat the protons, which of course everybody loses. But they are pretty insane collections of magnets, vacuum equipment. And once in a while a proton will pass through.
And then there's the spallation source itself. So this is what the target looks like. There's going to have to be liquid metal cooled in. And then out of here come lots and lots and lots of neutrons. Enough neutrons that you still need hot cells to deal with things. They're still quite radioactive, inactivated. But it's not a reactor. Other ways of making neutrons.
Speaking of, has anybody seen the pulsed fusion source that we have down in Northwest 13? No? We have a pulse neutron source that you can come take a look at. It's an electrostatic fusion pulsed machine. There's a whole bunch of tritium and deuterium in this palladium sponge, what happens to hold hydrogen and its isotopes very well. And with a very quick pulse, you can have a tiny pulse fusion and generate about 10 to the 8th neutrons that actually is a push button neutron source. So if you want to see a neutron source beyond reactors, go down to the vault in Northwest 13 and ask to see that.
We did a quick experiment before trying to activate cell phones to see what was in them. We did not generate enough neutrons to do so. But this cell phone has definitely seen a few fusion neutron pulses. And we checked later, it's not giving off any residual radioactivity. At least we can measure. That was a fun failed experiment.
And then comes the craziness. Since it's about five of, I want to get into things that will definitely not be on the exam. So just sit back and enjoy and stop taking notes.
Complete insanity can happen with super high energy electrons. We've already talked about Bremsstrahlung. We have talked about synchrotron radiation, where you have a charged particle going along a magnetic field line. It changes direction and gives off X-rays.
We haven't talked about inverse Compton scattering. Interesting process here. In comes a low energy photon, hits an electron, out comes a higher energy photon. Compton scatterings usually think of as the other way around, where a high energy photon comes in, scatters off an electron, loses energy. In this case, a high energy electron colliding with a low energy photon can impart energy to the photon. And you can actually calculate-- or in this case, I've just taken from a paper-- the energy gain from inverse Compton scattering, as well as whatever cross-section there is. And even though this is a very infrequent process-- well, the universe is pretty big, and contains a lot of things that have magnetic fields like stars and black-- whatever else happens to have magnetic fields.
And you can identify radio sources by looking for these inverse Compton scattered X-rays. So the Chandra X-ray map, I believe a piece of which or a receiver for which is up in the building in Porter Square. If you guys go down three stops on the red line, you'll see this little area full of Japanese noodle shops, Lesley University, a bunch of galleries. And in a little-- I think it's still there-- and a little sign that says, oh, and there's the Chandra X-ray Observatory. Whatever. They may or may not have moved, but I recommend you check it out.
And then what happens to those electrons? Well, they can actually decay. Pretty interesting things. And so some of this inverse Compton scattering has gone into the evidence for or against where cosmic rays come from, because you should see electrons of a certain energy after undergoing this process. I think I will skip ahead. Oh, I won't skip ahead.
And so what these cosmic rays can produce is what's called positive, negative, or neutral pions. Other subatomic particles with masses somewhere between protons and electrons that themselves can undergo different reactions or different decays into muons. And don't worry, muons and pions and such are not part of the topic of this course. But they do have known lifetimes, they do have known masses and charges, they do have known stopping powers. We should be able to measure them.
And there is a cosmic muon detector at Boston University. Or rather, it's a pair of detectors that looks for this coincidence of one muon scattering off one detector, or interacting, and another particle being sensed directly beneath. So we can actually sense these muons to confirm or refute the theories about where they come from in terms of cosmic rays.
And these neutral pions are what end up creating these gamma rays. I think they were around the 70 something MeV range. So if these theories about them are correct, we should be able to sense these gamma rays, and sense how many of them there are as a function of energy. That's not what I wanted to do. Let me recreate presentation mode, because this is definitely delving into the kind of stuff that, well, I'm not an expert in.
So a quick detour into subatomic physics. You guys probably have heard of that protons and neutrons are not the smallest building blocks of matter. They themselves can be composed of quarks and antiquarks with different charges and different masses. They're given different flavors. I don't know who came up with this terminology, but it's kind of fun. Things get kind of whimsical when you get into subatomic physics. And these quarks and the gluons between them are what composes protons, neutrons, in their antimatter counterparts.
And these sorts of things can also undergo their own decay and reactions. So when beta decay occurs, it's actually one of these up quarks turning into a down quark and releasing an electron and an antineutrino. But again, we're not going to delve even deeper into this.
There are other particles composed of other arrangements of quarks. So if you have just an up and a down, you have a positive pion. Which should have a charge-- I think one's 2/3 of one's minus 1/3. Yep, that is correct. So a plus pion should have a charge of 1/3 the charge of an electron. So if you know the mass of some particle because you know the number of quarks, and you know the charge of it, you should be able to calculate its own stopping power. And figure out how many should get through the atmosphere and such, or how many get absorbed in your detector.
And so when a very high energy proton collides with an atmospheric molecule, it creates neutrons, creates a shower of pions. These neutral pions-- much like electron positron interaction-- can produce their own gammas. So they can spontaneously decay from particles that are mass into gamma rays of pure energy. Which then go on to create their own shower of electrons and positrons by pair production. Because as we saw, the higher in energy you go for photons, the more likely pair production becomes. And there you have it. This is part of the 22.01 stuff, but taken to the nth degree.
And then the evidence for pion decay comes from extremely fine measurements of the number of these pions as a function of energy. Or in this case, look at that. They're-- what is it-- ergs per centimeter squared per second. What does that unit physically mean? Not going to get into that.
But at any rate, there are different models for how many of these pions or their high energy gamma decay products should be observed. And by looking at those very carefully, you can tell where they came from. Should they have come from coronal mass discharges, so we should know what energy those protons should be, or some other source
But I am going to stop there, because it is five of. So after that crazy detour, give you guys 10 minutes to degas and absorb some neutral pion gamma decay products. We'll meet upstairs in 10 minutes for an exam review.