Flash and JavaScript are required for this feature.
Download the video from iTunes U or the Internet Archive.
Topics covered: Valence bond theory and hybridization
Instructor: Catherine Drennan, Elizabeth Vogel Taylor
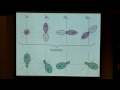
Lecture 15: Valence Bond Th...
Related Resources
Lecture Notes (PDF - 1.0MB)
The following content is provided under a Creative Commons license. Your support will help MIT OpenCourseWare continue to offer high quality educational resources for free. To make a donation or view additional materials from hundreds of MIT courses, visit MIT OpenCourseWare at ocw.mit.edu.
PROFESSOR: OK, I want to take 10 more seconds now the clicker slide. This is giving us one more try on the vsper geometries, because it didn't go so well on Wednesday. All right, excellent. So that is a very good job. Let's quickly go over why.
We have p h 3. We told you that phosphorous has 5 valence electrons plus 3 from each of the hydrogens, so we have a total of 8 valence electrons. How many do we need to get full valence shells everywhere?
STUDENT: [INAUDIBLE]
PROFESSOR: 14. So, we need 14 minus 8. That leaves us with 6 bonding electrons. And if we put that in our bond here, we have 1, 2, 3 bonds, plus we have one lone pair left over. So this is our Lewis structure here. If these bonds were all completely of equal distance apart, whether is was a lone pair or bonding electrons, the angles would be 109 . 5 degrees. But because there's this lone pair here, it's pushing down on the other bonds, so we end up with an angle of less than 109 . 5 degrees.
All right, so let's switch over to notes for today. So we're going to finish talking about molecular orbital theory, and then we'll switch over to discussing bonding in larger molecules, even larger than diatomic, so we'll move on to talking about valence bond theory and hybridization. So, clearly you don't have your notes in front of you yet, so you can just listen, take it all in. What I'll do is I'll post the notes filled in to the point where you actually get your class notes today. So, this will be a little bit more like a seminar to start with, and a little bit less like a lecture in class. But let's go ahead and start our discussion in terms of molecular orbital theory.
So where we had left off with was we'd fully discussed up to the point of considering homonuclear diatomic molecules, so molecules that both have the same nucleus. And where we had left off was we were going to start one example of thinking about now where we have a heteronuclear diatomic molecules, so two different atoms in terms of forming the molecule.
But first, I just want to remind you when we're talking about molecular orbital theory, this is treating electrons as waves, so what we're actually able to do is either constructively or destructively combine atomic orbitals to form molecular orbitals. So you should remember that any time we combine 2 s orbitals, what we're going to find is if we constructively interfere those two orbitals, we're going to form a bonding orbital. And that's going to be lower in energy than the two individual atomic orbitals. And we call that, for this case, our sigma 2 s orbital.
In contrast, if we have destructive interference, what we're going to form is a sigma 2 s star, and what does the star designate?
STUDENT: [INAUDIBLE]
PROFESSOR: Anti-bonding, yup. So it's an Anti-bonding orbital. It's going to be higher in energy than the individual atomic orbitals.
All right, great. So, I think we have these molecular orbital energies down, so let's move on to talking about more complex molecules. And to do this we're going to introduce valence bond theory, and the idea of hybridization of orbitals. So the idea behind valence bond theory is very easy to understand. Essentially what you have is bonds resulting from the pairing of unpaired electrons. So the simplest case we can think of is with h 2 where we have two unpaired electrons, each in a 1 s orbital of a separate h atom. And if we picture those two coming together, we form the h 2 molecule. And again, we have the pairing of the unpaired electrons, and we have two orbitals coming together.
So in molecular orbital theory, what we did was we named orbitals based on their symmetry. In valence bond theory, the focus is on discussing the bonds, but it should look very familiar to you, because there's two types of bonds that we want to discuss here. We want to discuss sigma bonds and pi bonds. So this is very similar to what we saw in terms of sigma orbitals and pi orbitals.
So in this first case here, what we're seeing is a sigma bond. And a sigma bond forms any time you have two orbitals coming together and interacting on that internuclear axis. So we talk about a sigma bond as being cylindrically symmetric about the bond axis, and it's important to point out that it has no nodal plane across this bond axis.
This is in direct contrast to when we're thinking about pi bonds. So pi bonds have electron density both above and below the bond axis, but they actually have a nodal plane at this z, this bond axis here. And remember for this class, we always define z as the internuclear or the bond axis.
So it might look like here, if you don't understand about p orbitals, which I know all you do, but if someone else was just looking and seeing, it kind of looks like there's two bonds here. There's not two bonds, that's one pi bond, and the reason is because it's 2 p orbitals coming together, and remember p orbitals have electron density above and below the axis, so when they come together, it kind of looks like one bonds, but essentially what we have here is one pi bond.
So let's think about how we can classify single and double and triple bonds, which is what we're really used to dealing with in terms of these sigma bonds and these pi bonds.
So, if we take a look at what a single bond is, and let me grab some molecules here. If we're talking about a single bond, we're talking about 2 orbitals overlapping in the internuclear axis. So if we have a single bond here, would you consider that a sigma bond or a pi bond?
STUDENT: [INAUDIBLE]
PROFESSOR: Right, it's a sigma bond. Essentially what we're seeing is overlapping in this z axis here.
In contrast, if we talk about a double bond, what we're now talking about is having both a sigma bond and also one pi bond. And I apologize, I intended to set this up right before class, but that didn't happen today. All right, so what we see here is we have our sigma bond that's along the internuclear axis here, but we also have a pi bond, because each of these atoms now has electrons in it's in a p orbital, so we're going to overlap of electron density above and below the bond. So that's exactly what our definition of a pi bond is, so we have one sigma bond, and one pi bond.
So now let's think about a triple bond. A triple bond, again is going to have one sigma bond on the internuclear axis. How many pi bonds would you expect?
STUDENT: [INAUDIBLE]
PROFESSOR: Two, great. So, we're going to see two pi bonds. The first one will be above and below the bond axis is where we'll see the electron density, and the second will be perpendicular to that, so it will be a density in front of and behind the bond axis. So we can kind of flip it this way -- this will be one pi bond, this will be another interacting between these p orbitals.
All right, so that's really all there is to thinking about valence bond theory in terms of the most simple explanation here. But what we're going is we're going to start trying to apply it to a molecule, and I actually picked a molecule that it's not going to work for, even though it would work even just at this level for many, many molecules. And I picked looking at methane so we could see if there are other factors that we're not considering, that we need to maybe tweak our model a little bit, and I think we'll find that we do if we take a look at a polyatomic molecule, methane, so c h 4.
So let's think about methane using valence bond theory. So, using our simple valence bond theory, what we would expect is that we want to pair up any unpaired electrons in methane with unpaired electrons from hydrogen and form bonds. But what we see we have is that we only have two unpaired electrons here. Because we have paired set in a 2 s orbital, so all we're left essentially is two electrons that are available for bonding. So this should immediately look like a problem because we know, in fact, that methane is tetravalent, and this is telling us it's only divalent. Essentially it would only allow for us to bond to two hydrogen atoms. So if it did this, it now looks like, from looking at the paired electrons that we have a stable structure here, and our structure is not c h 4, it's a stable structure of c h 2, and it will actually predict, also, what this h c h bond angle it is. So according to this model what is that bond angle?
STUDENT: [INAUDIBLE]
PROFESSOR: One more time. OK, I hear a mix.
So, according to this model, what we're seeing is a bond angle of 90 degrees. What do you know the bond angle should be? It's 109 . 5 is what we would expect for methane because it's tetravalent, but here we're just seeing something that's divalent, and they're both in p orbitals that are perpendicular to each other. So what we're predicting is a bond angle of 90 degrees. This is totally wrong, this is the wrong picture altogether. If you had your notes, you could do some fun scribbling right now, so you can do that at home. We're going to need to tweak our explanation here, and take into account another factor, and that factor is the fact that we know that we must have four unpaired electrons in carbon if we're going to form four bonds.
So the way that we can explain this is through something called electron promotion and hybridization of atomic orbitals. So let's take a look at what we mean by this. So if we take our carbon atom here, which has two electrons in the 2 s orbital, and we promote one of these electrons into a 2 p orbital, what we see now is that yes, we do, we have four unpaired electrons.
So, looking at this, this might not look so good for you. What we're proposing here is that you take a nice low energy s electron and move it into a higher energy p orbital. And the truth is that yes, this costs energy, we're going up to a higher energy state. But it doesn't actually cost as much energy as you might think, because in this s orbital here we have a paired electron situation where we're moving up to a p orbital where the electron is no longer paired, so it won't feel quite as much electron repulsion, but nonetheless, this is going to cost us energy. So we'll have to think about where that energy is going to come from and we'll see that in just a minute.
But let's assume that this is, in fact, going to happen. So now what we have is four unpaired electrons. That's great, but it's still not quite the picture we need, because actually, all the electrons are not in equal orbitals -- one's in an s orbital, and 3 are in p. But what we need to remember is the fact that we're talking about electrons which are waves.
When we're talking about orbitals, we're talking about wave functions. So we can actually constructively and destructively combine these waves, these atomic orbitals to make a hybrid. So if we go ahead and hybridize our p orbitals and our s orbitals, we'll switch from having these original orbitals to having something called hybrid orbitals. And hybrid orbitals are all going to be completely equal, and you'll notice that they're higher in energy than the s orbital, and lower in energy than the p orbital. That should make sense because they come from combining s orbitals and p orbitals. And specifically, when we give them a name it's very clear exactly which orbitals they come from combining, we're calling these s p 3 orbitals -- that's because they come from combining 1 s orbital and 3 p orbitals.
You should never get the names of hybrid orbitals wrong because it's very straightforward. If it has 1 s and 3 p's, we call it s p 3. Naming doesn't always make sense in chemistry, so I like to point out this is a place where naming does make a lot of sense.
All right, so let's consider our methane situation now that we have our hybrid orbitals. So I want to mention also, these are exactly equivalent, they're equivalent in energy, they're equivalent in shape. The only thing that is different about these orbitals is their orientation in space. So actually, first let's take a look at how we got these orbitals. We got them from combining again, 1 s orbital and the 3 p orbitals. If we hybridize these, what we end up seeing are these four hybrid orbitals. You'll notice the shape is the same, all that's different is their orientation.
So essentially, each of these orbitals come from linear combinations of all of the original orbitals, and it's hard to picture exactly how that happens, but one that you can at least start to get an idea is if you think about combining the 2 s and the 2 p z here, which is not quite accurate because of course, we're combining all of them. But it would just give you a little bit of an idea of shape. You can see if we combine the s with the top lobe of the p, they're going to constructively interfere because they have the same sign. So you see in the hybrid orbital we actually have a larger lobe on top where they constructively interfered.
If you compare the s orbital with the bottom lobe, these have a different sign so they're going to destructively interfere. So what you see is actually a diminished lobe on the back part of this s p 3 orbital. So s p 3 orbitals always have one huge lobe and one really little lobe. A lot of times when people draw them, they even only draw the big lobe just to keep their paper looking nicer, but there is that little tiny lobe on the other side.
All right, so in terms of s p 3 hybrid orbitals, let's combine all four together on one axis, because this is what's going to happen in an s p 3 carbon atom. So in this case, what would you say that the angle is here?
STUDENT: [INAUDIBLE]
PROFESSOR: Right, great. So, we've achieved the angle that we observed, which is good, which is a 109 . 5. So we can think about now doing bonding, and now we have four equal orbitals with one electronic each. So we can bring in four hydrogen atoms, which will each contribute another unpaired electron. So now what we have is four bonds.
And we can think about where we did get that energy for electron promotion that I mentioned before where we moved the electron from the 2 s to the 2 p. We get that from bonding. We're going to release a lot of energy for bonding, it's going to more than make up for the fact that we actually had to spend some energy to promote that electron.
So, we can think about now how do we describe this bond in valence bond theory. So the way that you describe a bond is you describe the orbitals that the bond comes from, and also the symmetry of the bond. So would you expect this to be a pi bond or a sigma bond here?
STUDENT: [INAUDIBLE]
PROFESSOR: OK, so I'm hearing some mixed answers. It turns out that it's a sigma bond. The reason that it's a sigma bond is because the s p 3 hybrid orbital is directly interacting with the 1 s orbital of the hydrogen atom, and that's going to happen on the internuclear axis, they're just coming together. Any time two orbitals come straight on together in that internuclear axis, you're going to have a sigma bond. So if we go ahead and name this bond, what we're going to name it is sigma, because that's the -- basically the shape of the bond or that's how our bond is coming together. And then we're going to name the atomic orbitals that make it up, and it's being made up of a carbon 2 s p 3 orbital, and a hydrogen 1 s orbital.
All right, so let's think of a case now that's getting a little bit more complicated. We were talking about methane, which has only one central atom. We can also talk about atoms that have two or more central atoms. So let's talk about ethane now, which is c h 2. So let's take our carbon s p 3 hybridized carbon and just move it around here so we can make the z inter- bonding axis between the two carbons right here. So if we still have an angle of a 109 . 5 degrees, and again, we still have four unpaired electrons available for bonding, we can make one of those bonds with another s p 3 hybridized carbon, so we're going to make up one pair here. If we think about that, that's a sigma bond, right, they're coming together along the nuclear axis.
We also have six spots available to form hydrogen bonds, so we can go ahead and fill in those electrons as well. So in terms of thinking about ethane, we actually have two bond types that we're going to be describing just in terms of the carbon-carbon bond and then the carbon h bonds.
So let's talk about ethane and how we would actually write these bonds. If we have the molecule ethane, then what we're going to have first is our sigma bond that we described between the two carbons. So it's going to be carbon, and then what's the hybridization here?
STUDENT: [INAUDIBLE]
PROFESSOR: All right, start again, what's the hybridization of the carbon atom?
STUDENT: [INAUDIBLE]
PROFESSOR: OK, so it's 2 s p 3, and our second carbon is also 2 s p 3.
All right, so this is our first type of bond here. Our second bond is going to be between the carbon and the hydrogen atoms. Is that a sigma or a pi bond?
STUDENT: [INAUDIBLE]
PROFESSOR: Sigma, good. So again, our carbon is going to be 2 s p 3. And what will our hydrogen be? 1 s -- we don't have to hybridize it, it already has only one unpaired electron in a 1 s orbital. All right, so that's how we describe ethane.
We don't have to just stick with carbon, we can think about describing other types of atoms as well using this hybridization. For example, we can talk about nitrogen, and nitrogen has five valence electrons shown here. Would you expect to see electron promotion in nitrogen where we pull one of these 2 s electrons into one of the 2 p orbitals?
STUDENT: [INAUDIBLE]
PROFESSOR: No, good. So, electron promotion does not happen in terms of nitrogen, because it would not increased our number of unpaired electrons. No matter what we do in terms of promotion, we're always going to have three unpaired electrons. We can still hybridize all these orbitals, however, so we can still form four hybrid orbitals, which are again, 2 s p 3 hybrid orbitals.
So if we take a look at nitrogen here, what you'll notice is we have thre available for bonding, and we already have our lone pair -- one of our orbitals is already filled up. So we can add three hydrogen atoms here, and fill in our other orbitals right here. So if we do this and we form the molecule ammonia, let's switch to a clicker question, and have you tell me what the bond angle is going to be in ammonia -- the h n h bond angle. Actually, let me draw it on the board as you look -- actually, can you put the class notes on, since you don't actually have your notes to refer to. So there's the class notes there.
All right, this should be a pretty quick thing for you to figure out, so let's just take 10 seconds on this. OK, great. Even thinking quickly, most of you got it correct. So what we see is on ammonia here, we know that it's less than a 109 . 5, it's actually 107, so it's less than a 109 . 5, because of that lone pair pushing down in the bonding electrons. And what is the shape, for one more clicker question on ammonia?
Let's take 10 seconds again, this should be pretty quick. All right, pretty good. So, 70% of you. We'd like to get this up higher. The shape is actually trigonal pyramidal. And you need to just remember your shapes. If they're not obvious to you what they're called, you need to just study them and learn them. So it's trigonal because we have these three atoms that are bound to the central atom here, and if you picture it, it's actually shaped like a pyramid. So it's trigonal pyramidal. That's what we call when we have three bonding atoms and one lone pair.
All right. So we can switch all the way back to our notes here. And the last thing we can think about is how do we name this n h bond, and again, we just name it based on it symmetry. It's a sigma bond, and it's going to be -- no. OK, it's going to be nitrogen 2 s p 3, because it's a nitrogen atom, and then hydrogen 1 s. So, I don't even have to worry because you're not writing this down, so I can just fix it when I post the notes and no one will ever know, except that this is not OpenCourseWare.
So let's switch to thinking about oxygen hybridization here. So in oxygen we have a similar situation where, in fact, we are not going to promote any of the electrons because we have two lone pair electrons no matter what we do. So when we hybridize our orbitals, we're going to end up with again, four hybrid orbitals, 4 s p 3 orbitals, and what we'll see is that two of these are already going to be filled up with a paired electrons, so we're only going to have 2 orbitals with an unpaired electron available for bonding.
So let's think about water here as our simplest example with oxygen. So we can have our two hydrogen atoms come in here, and what we will find is now that we have all of our orbitals filled up -- so thinking about what this angle is here, would you expect it to be less than or greater than what we saw for ammonia before?
STUDENT: Less than.
PROFESSOR: Good, good, it's going to be less than, and it's going to be less than because now we have two lone pairs. So since we have two lone pairs, we're going to be pushing down even further on the bonding electrons, so we're going to smoosh those bonds even closer together. The bond, it turns out, is 104 . 5 degrees, that h o h bond.
So in terms of naming our o h bond, good, it's right here. So it's going to be a sigma bond, and we have oxygen 2 s p 3 and hydrogen 1 s. And the geometry, which I didn't ask you, is going to be bent for this molecule.
All right, so that's s p 3 hybridization, but those aren't the only type of hybrid orbitals that we can form. Let's take a look at what happens if instead of combining all four orbitals, we just combine three of those orbitals, and what we'll end up with is s p 2 hybridization.
So in s p 2 hybridization, instead of combining all four, we're just combining two of the p orbitals with the s orbital. So what we're going to end up with now is three hybrid orbitals. And what happens to this last p orbital is nothing at all, we just get it back. So we end up with 1 p orbital completely untouched, and three hybrid s p 2 orbitals.
So again, we can think of an example here. So let's take boron, for example, and this has -- it starts off with three valence electrons. Would you expect to see electron promotion for boron?
STUDENT: Yes.
PROFESSOR: Yeah, absolutely. if we move up one of our electrons into an empty p orbital, what were going to see is now we have three unpaired electrons that are ready for bonding.
So, if we hybridize just these three orbitals, what we're going to end up with is our s p 2 hybrid orbitals. Again, the name is very straightforward, it comes from 1 s and 2 p orbital, so it will be s p 2. And again, you might be thinking well, why didn't we actually hybridize this 2 p y orbital. It doesn't actually have an electron in it, so we don't have to worry about whether it's very high in energy or not, we don't care that it's high in energy. What we do care about is the energy of our orbitals that have electrons in them, and if we combined all four of the orbitals, then our hybrid orbitals would have more p character to them, so they'd actually be higher in energy. So if we don't have to hybridize one of the p orbitals, we can actually end up with a lower energy situation, because now these s p 2 orbitals are 1/3 s character, and only 2/3 p character, instead of 3/4.
So we end up with 3 s p 2 hybrid orbitals, so we can think about what would happen here in terms of bonding, and if we think about how to get our bonds as far away as possible from each other, what we're going to have is the trigonal planer situation. So if you picture, for example, b h 3, it's going to look like this. All of our electrons are in our bonds, we want to got them a 120 degrees away from each other, that's as far away as we can get them.
Keep in mind we do have this p orbital here and it's coming right out at us. And this p orbital is here, but it's empty, it doesn't have any electrons in it, that's why we don't have to worry about it in terms of getting our electrons as far away from each other as possible.
So what we'll have here is a trigonal planar case, and you can see that we only have three electrons that are set for bonding, so we'll add three hydrogens, and for b h 3, we'll get a stable structure here.
So, remember, boron was one of those exceptions to our Lewis structure rules where it was perfectly happy not having a full octet. So this can tell you why it's so happy with only having six electrons around it.
All right, so if we think about b h bond here, again, it's the sigma bond, and we're going to say it's a boron 2 s p 2 hybrid orbital interacting with a hydrogen 1 s orbital. So let's take a look at another case where we have s p 2 hybridization, we can actually also have it happen in carbon. So if we think about having it happen in carbon, we're starting with the situation where we've already promoted our electron into a 2 p orbital here, and what we're going to do is just combine the s and two of the p's, so we'll end up with electrons in one of each three s p 2 hybrid orbitals. But unlike the case with boron where we had an empty p orbital, we're actually going to have an electron in the p orbital of carbon as well.
So again, if we think about that shape of that carbon atom, it's going to be trigonal planar, it's going to have bond angles of 120 degrees, because we have this set up of having three hybrid orbitals.
So let's take a look at what actually happens if we're talking about a carbon-carbon double bond, such as in ethene, c 2 h 4, we're going to have a double bond. If we have a double bond, we know we need to have only one sigma bond, and we're also going to have one pi bond. So it already should make sense why we have that p orbital there, in order to form a pi bond, we're going to need a p orbital. So if you picture this as our s p 2 carbon atom where we have three hybrid orbitals, and then one p y orbital coming right out at us. So again, we picture the same thing as we pictured with the boron there. If we have, coming along this z axis, another carbon atom, we can actually form one bond between the two carbon atoms there.
So if we picture how this happens, what we have here if these are our 2 s p 2 carbon atoms -- so here we have s p 2 hybrid carbon, and here we have s p 2 hybrid carbon atom. These 2 are going to come together like this, and the first bond that we're going to form is going to be a sigma bond, right, so we see that here. If we're looking head on, we see they form a sigma bond. We can also look at them coming in from the side, and that's what I tried to depict here where you can actually see in pink is the p orbital. So we can also show them coming together this way, so now you're looking at it where you can see the p orbital, and maybe just see well one of the hydrogen atoms.
So we can have four total hydrogens bonding here, and we can think about how to describe these carbon-carbon bonds. So in the first case of this first bond here that I've put in a square, what type of a bond is this, is the sigma or pi?
STUDENT: Sigma.
PROFESSOR: Yup, it's a sigma bond. We're having two orbitals coming together on the bond axis. So we'll call this sigma, and it's between two s p 2 hybrid carbon atoms. So it's stigma carbon s p 2, carbon s p 2.
What about this second bond here where we're going to have interaction of 2 p orbitals, is that sigma or pi?
STUDENT: Pi.
PROFESSOR: Pi, great. So our second bond is going to be a pi bond. And again, this is between the p orbitals, these are not hybrid orbitals, so when we name this bond we're going to name it as a pi bond here, because it's between two p orbitals, and it's going to be between the carbon 2 p y orbital, and the other carbon 2 p y orbital. Remember, we didn't hybridize the 2 p y orbital, so that's what we have left over to form these pi bonds.
All right. So in addition to having these two carbon bonds, we actually also have four carbon hydrogen bonds in addition to our carbon-carbon bonds. So why don't you tell me what the valence bond description would be of these carbon hydrogen bonds?
So let's take 10 seconds on that. OK, great. So most and you got it, so we can switch to the notes and let's talk about this here.
So in terms of the carbon hydrogen bond, it's a sigma bond, because we define it -- any time we are bonding to an atom, we have to keep redefining our bond axis to whatever two atoms we're talking about. So it's along the bond axis and it's between a carbon s p 2 hybrid, and then the hydrogen is just a 1 s orbital that we're combining here. So those are our three types of bonds in ethene.
One thing that I want to mention that is really important is once you have double bonds, what happens between those two atoms in the molecule is they can no longer rotate in relation to each other. So you can think about why that is. When we have just a single bond in them molecule, you have all the free rotation you want, you can just spin it around, there's nothing keeping it in place. But once you have a double bond here, we have our pi bond, as well as our sigma bond.
So there's electron density above the bond and below the bond. So if I try to rotate my 2 atoms, you see that I have to break that pi bond, because they need to be lined up so that the electron density can overlap. So in order to rotate a double bond, you have to actually break the pi bond, so essentially what you're doing is breaking the double bond. So really, you can not ever rotate a double bond, it makes your molecule very rigid.
This is incredibly important because if you picture having a double bond in a very large molecule, you could have all sorts of other atoms off this way and all sorts of other atoms off this way, and you can picture the shape would be very different if you have one confirmation versus another confirmation. So it's very important that the double bond locks it in a particular conformation. This completely could change if you were to flip from one to the other conformation which can happen in chemical reactions. If you were to make that change you would find that the molecule now has completely different biological and chemical properties. So it's very important to be keeping in mind that any time you see a double bond, you have a pi bond there, so you're not going to see any rotation around the bond axis.
All right, so let's think of a more complicated example of having a double bond, and maybe a more interesting example, and this is talking about benzene. I think most and you have talked a little bit about benzene over this past week in recitation. Benzene is a ring that's made up of six carbon atoms and six hydrogen atoms. So let's picture what this looks like here, and we'll start with four and we'll add in our last two.
So essentially, we have two ethene or ethylene molecules here to start with where these blue are our 2 s p 2 hybrid orbitals, so you can see that for each carbon atom, one is already used up binding to another carbon atom. If we think about bringing in those last two carbons, what you can see is that for every carbon, two of its hybrid orbitals are being used to bond to other carbons. So that leaves each carbon with only one hybrid orbital left. And if we think about the six hydrogens, now each of those are going to bind by combining one of the carbon hybrid orbitals to a 1 s orbital of hydrogen.
So, if we think about what bonds are in this molecule, we actually have six of these sigma carbon s p 2, carbon s p 2 bonds. We also have carbon s p 2 hydrogen 1 s bonds. How many of those do we have? Yup, we also have six of these, because we have six carbon hydrogen bonds. So that's two of our types of bonds in benzene, and we have one type left, and that's going to actually be the double bond or the pi bond that forms between some of these p orbitals.
So we can have one bond here between this carbon's p orbital and this carbon's p orbital. So let's have a clicker question here on how many total pi bonds do you expect to see in benzene? Oh good, so it's left up -- the notes are left up on this screen right now.
All right, so let's take 10 seconds on that. All right, great. So, most of you saw that what we would expect to see is a three bond, some of you thought six. So let's take a look at why three is correct. So, what we end up having is three of these pi -- 2 p y 2 p y bonds, we can have one between these two carbons here. Can we have one between these two carbons here if we have one here?
STUDENT: No.
PROFESSOR: No, we can't. We're already using it up in this pi bond here, so that means we're limited to only two other spots on the molecule, so we have three. But, of course, what you saw in recitation, and hopefully, what you can now think very quickly by looking at this, is that this is not the only configuration of pi bonds that we could have in benzene. There's absolutely no reason I couldn't have switched it around and said that instead the pi orbitals form between these atoms instead of those first atoms I showed.
So, let's look at this in a more simple structure here where we have the two possible forms of benzene, and the reality is is that it's going to be some combination of the two. This is resonance, this is a case of a resonance structure. So what we see is that those six pi electrons are actually going to be de-localized around all six of those atoms. So if you think about any one of these carbon-carbon bonds, what type of a bond would you expect that to be? What would the bond order be for this bond?
STUDENT: [INAUDIBLE]
PROFESSOR: Yup, it's going to be a 1 and 1/2 bond. It's a 1 and 1/2 because it's halfway between a double bond and a single bond. So, of course, this is resonance so we can go ahead and put our resonance notation in there to indicate that benzene is a resonance structure.
All right. So let's quickly talk about our last type of hybridization that we're going to discuss today, which is s p hybridization. So s p hybridization comes now from when we're combining an s orbital now with only one p orbital. So let's take a look at this with carbon. And if we hybridize these orbitals in carbon, what we end up with is having two hybrid orbitals, and then we're going to be left with two of our p orbitals that are each going to have an electron associated in them. So again, looking at the shapes, now we're just combining two, we've got these two equal hybrid orbitals plus these 2 p orbitals here.
So let's take the case of acetylene where we have two carbon atoms that are going to be triple bonded to each other, each are bonded to a carbon and then to one hydrogen. So this is a little bit trickier to look at and see what it means, but essentially we have two hybrid orbitals, which are shown in blue here, and then we have one p orbital that's left alone that's going up and down on the page. And then that second p orbital's actually coming right out at you, it's coming out of the screen at you.
So, if we think about this z bonding axis between the two carbon atoms, we can picture overlap of those s p hybrid orbitals, and then we can also picture bonding to hydrogen. So, in a settling, what is the bond angle here? This is the easiest question all day, what is the bond angle between all of these? Great, so it's 180 degrees.
So, if we think about the bonds that are forming -- oh I see our TAs are here, so you can start handing them out, because we have two minutes left to go.
So, as they're very quietly handing out your class notes, let's think about what this bond is here, this boxed bond, is it a pi bond or a sigma bond? It's going to be a sigma bond. So, we have sigma 2 s p, carbon 2 s p. So they're two s p bonds combining.
Now let's think about this first pi bond, which will be above and below the bonding axis. Is this pi or sigma? This is pi. So we're talking about pi carbon 2 p x, because it's the x axes combining to carbon 2 p x.
And the last bond that we have here is a carbon-carbon bond, and this is our last p orbitals that are coming together. These are the ones that are coming right out at you, so this is going to be on a second pi orbital. So this will be pi carbon 2 p y, carbon 2 p y.
All right. So, we'll stop here today. Just stay in your seats for another 30 seconds as they're handing out your notes. I want to mention that you're going to get problem-set five is posted today, and I'll write which ones you can already do so far, because you don't have class on Monday. But remember that you do have recitation on Tuesday, so that could be very helpful with the problem-set, so be sure to go to recitation on Tuesday, and have a great long weekend.
Free Downloads
Free Streaming
Video
- iTunes U (MP4 - 87MB)
- Internet Archive (MP4 - 91MB)
Caption
- English-US (SRT)