This resource is available as audio only.
Flash and JavaScript are required for this feature.
Download the track from iTunes U or the Internet Archive.
Description: This lecture covers fixed action patterns as well as how these innate behaviors are organized in the central nervous system (CNS).
Instructor: Gerald E. Schneider
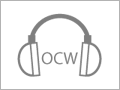
Lec6: Fixed action patterns...
The following content is provided under a Creative Commons license. Your support will help MIT OpenCourseWare continue to offer high-quality educational resources for free. To make a donation or view additional materials from hundreds of MIT courses, visit MIT OpenCourseWare at ocw.mit.edu.
PROFESSOR: We have a few more slides from last time to finish up because of the problems I had with the computer. We have a class Wednesday with a quiz. And Friday, as most of you know, it's a student holiday. I would encourage all of you to go to the career fair-- especially if you've not been to one before. Even if you fully expect to go to medical school or something like that-- you never know. You should know what's out there.
All right we started talking about the ethology of geese last time, and we saw a video where you could see Konrad Lorenz and some of his early work discovering imprinting. They showed the work he'd done with geese. And then later, work on social behavior of geese and the role of early experience and learning.
What was the answer to this question-- "Is the response of geese to an aerial predator innate?" In the video you saw this bird shape-- it was actually made to look quite a bit like a bird in that video, but you can use a silhouette-- that they glided above the birds. And the birds showed a mobbing reaction. They forget about their individual squabbles and whatever they had been doing, and they all pretty much do the same thing. And they protect their young and so forth.
Is that an innate response? What did we see? Did they have an innate response to the shape of a hawk as opposed to a goose? Hawk shapes-- short neck, longer tail. The goose shape-- the duck shape-- long neck, shorter tail. So they make a very different silhouette when it's moving. Is that an innate response?
There's still some claims in the literature that in perhaps some birds it is innate. And it's clear there are some innate responses made to overhead movement. But the precise shape they're responding to is probably not innate.
That doesn't mean that birds don't have many innate responses to specific visual configurations, because they certainly do. Migrating birds, for example, can respond to the patterns of the stars. But in this case they're responding to slow, overhead movement, and the species may differ a little bit into the details of the movement they respond best to. But that doesn't mean it doesn't change with experience. They do. Depending on their experience, they can change.
So that was this discussion. And this is the paper that did that-- a very nice analysis of the whole hawk/goose story-- because of some of the early work of Tinbergen and Lorenz, some of which they did together before World War II, where they met and spent the summer together working on this and other problems.
Let's talk a little bit about human instincts, or fixed-action patterns. We know that yawning can be considered a human fixed-action pattern. Tends to be triggered by the yawning in other people, and it seems to have some kind of social meaning. It doesn't only mean that you feel tired. In fact, it has other meanings as well. And there's still some things about it we don't fully understand. But we do know it's inbuilt.
These are their other examples. First of all, expressions of emotion. That was the work, remember, of Irenaus Eibl-Eibesfeld, where he studied emotional expressions in blind and deaf people. People born blind and deaf, found that they were the same as in normally sighted and hearing people.
And he also studied across cultures. He went to cultures that had never seen Western expressions at all. And people in those cultures made all the very same types of expressions that you see in what we call the more developed cultures.
Walking is an instinct. But don't we teach our children to walk? We always say that. "Oh, I've been teaching my child to walk, and he finally did it. He was a little bit slow to learn." Is this an accurate way of talking? Somebody?
No. Because walking is instinctive behavior. The child starts walking because his nervous system has reached the state of maturity where the circuits underlying walking are mature enough. They're spinal circuits, primarily. Also hindbrain. That's what you need. You need the balance of a vestibular system and so forth, which is a hindbrain sense. But it's instinctive movement.
Then I said that eye blinks and swallowing are actually fixed-action patterns, so they're normally called reflexes. So why would they be called fixed-action patterns and not reflexes? What do they need to be a fixed-action pattern and not a reflex? That could be on the quiz on Wednesday. There's got to be a difference. Yes?
AUDIENCE: Fixed-action pattern has some type of motivations to it.
PROFESSOR: OK, there's some motivation which builds up over time. So the longer you go without an eye blink or without swallowing, the more likely you are to do it. And it's difficult to go too long without blinking your eyes. I mean, there's a clear function to that. You need to lubricate the corneas.
And we can stare at each other and see who blinks first, but in fact the motivation to blink-- the motivation goes up and up and up until the threshold for eliciting that response becomes very low, and almost anything can cause it.
Of course we blink our eyes to protect our corneas not just from drying, but from other things too. If you puff air on it, that's the one that's normally called a reflex any touch of the cornea will elicit. But it also fits all the characteristics of an instinctive movement, a fixed-action pattern
It doesn't normally respond to a sensory trigger, but it will occur with almost no trigger-- with very little trigger-- after enough time passed. And the same is true for swallowing. We don't swallow as often as we blink our eyes, but it still has many of the characteristics of a fixed-action pattern. And that we discussed a little bit here.
So now let's go to another term common in ethology-- the concept of a supernormal stimulus. We say such a stimulus acts as a releaser of a fixed-action pattern. And I'm asking here for a fixed-action pattern in herring gull chicks, but you can think of other kinds of supernormal stimuli for other animals.
So in the case of herring gulls, they have this conspicuous red orange spot-- pretty conspicuous-- you can see it there in the gull. That orange spot. It's seen better from below. And gull chicks respond to that spot when their parent is over them and moving.
You can get an even stronger gaping response just by painting an orange spot on a yellow pencil and moving it a lot. You can get a stronger response. You can make it a supernormal stimulus by making it more prominent, by more movement. You can have perhaps greater contrast. But you can elicit that response even more. When you create a stronger response than normal with a slightly different stimulus, then we call it a supernormal stimulus.
Another early discovery by ethologists was the supernormal stimulus in gulls to an egg that's rolled away from the nest, like a herring gull-- they nest on shore. An egg that rolls away from the other eggs, the bird reaches out and hooks his beak around it, and pulls it back into the nest by rolling it back in the nest. And if you make an egg larger than normal, he responds more strongly, shows more vigorous egg rolling. Serves as a supernormal stimulus.
So then I want some examples in humans. Can you think of any supernormal stimuli in humans? We actually use them, yes, in advertising and social behavior.
Let's start with foods. Foods that are especially sweet and high in fats serve as supernormal stimuli. We have an instinctive preference for sweeter and fatter foods. At least most of us do.
And I put in here beware of restaurants, because there's a simple reason why I say beware in restaurants. The restaurant owner is in business. He wants to sell more food. He wants to sell food that attracts people.
And the same is true of supermarkets. I say beware of supermarkets, too. I always read the label. For example, when I buy a jam, I read all these different jams that are lined up on the shelf. I read calories per tablespoon. 50 every one. And then you read the details and you find out why. They've added high fructose corn syrup to it-- one of the most common ingredients in the American supermarket is high fructose corn syrup. Why? Because it's sweet. Because people like it better when it's sweet.
And because I'm diabetic, I don't want the 50 calories. I want it to be natural. I don't want artificial sweetener either. So I prefer to make the jam myself, or find one that doesn't add that. They even will say "all natural" on it sometimes, and they'll still add sugar. They'll say, well, sugar's natural. Anyway, it's because it's instinctive that it's used to make more money.
Think of the stimuli in sexual attraction. We call it the "poster effect" in advertising. When they advertise cars or other products, they often use a beautiful woman in the ad. Why? Just to attract attention. They're trying to sell it. They put them on posters even if they have nothing to do with the product that they're actually advertising-- with a beautiful smile. That helps, too, because we have an instinctive response to that.
What about enhancements of appearance? How has male appearance been enhanced in men? Of course, we exaggerate shoulder width. We create suits, we put shoulder pads in. I mean, men already have broader shoulders than women on average, but to enhance that we add shoulder pads.
In medieval costumes, it was common to enhance the penis area by putting this cowl-- often in a different color. And you see it in Shakespearean plays if they have period customs.
In primitive tribes you often see-- for example, many of them use this-- sometimes the only clothing they wear is a penis sheath if they're males. Enhance the whole appearance-- usually of an erect penis. But it's just part of the costume. So another enhancement. It's creating a kind of supernormal stimulus.
Female, it's even easier to think of these things. The waist-to-hip ratio-- men have a natural response to young women with a certain waist-to-hip ratio. We'll talk about that when we talk about research in sociobiology. But girdles and bustles were invented in order to enhance that.
Of course, breast prominence-- that's still pretty common, especially in young girls-- you see it in high school. But it occurs almost everywhere in women of different ages.
Think of other aspects-- lip color. Why is lip color enhanced? Why do women wear lipstick-- and in fact they enlarge the lips sometimes. What is that related to? You don't know? You do it, right? Some of you do. You know why? You know, you don't have to know why. When it comes to instincts, you don't have to know why. It's built-in. You don't have to know where it came from. Well, in sexual arousal, lips become redder and larger. So it elicits this response-- especially from the opposite sex.
Why would shoulder size be enhanced in women? I can think of one possibility that would fit what we've been saying. And one is simply that the hourglass shape is not just waist-to-hip ratio, but it's the whole enhancement of a narrow waist by make the shoulders a little wider.
But I think there's other reasons in modern culture. In fact, it's become much more common in modern culture than it was many years ago. And that is that women want to de-feminize their appearance somewhat. They want to be more like men.
Now we don't think of it that way so much, but certainly when women first began to enter the business world a lot more. When did women really enter the world of business and the world of labor? During the wars, when all the men were going off to war. The economy would have fallen apart. So older men that were not going to the war, they needed help. So women came in and took over a lot of those jobs.
But now, of course, women are at every level in business-- including right up to the top. But it's still fairly common for a business woman to wear suits that look like male suits. All right, well, anyway that's a somewhat separate reason that has nothing to do with supernormal stimuli. It has to do with kind of imitation.
Then Scott in your textbook, he's talking about controlling behavior-- the role of the nervous system. And I would like you to at least know simple definitions of neurons and nervous systems so we can talk more easily about it. So I'm asking you for definitions of a primary sensory neuron, a secondary sensory neuron, a motor neuron, an interneuron.
How do we define those things? You should all know. You've all had 901. Or you're taking it now. But maybe you didn't learn it, because they're not always so attentive to neuroanatomical details as I am.
So let me give you some definitions. A primary sensory neuron is a neuron with a cell body outside the brain or spinal cord-- the central nervous system is brain and spinal cord-- the peripheral nervous system are outside. Peripheral nervous system are the nerves going to our skin and muscle.
So the primary sensory neuron has the cell body outside. In primitive animals, it's in the surface epithelium. In us, it's in a ganglion near the central nervous system with a peripheral axon going out to the periphery. We have them in a little bit different shape for the skin of the body and for, say, the auditory or the olfactory system. But all these systems have a primary sensory neuron.
What is a secondary sensory neuron? Well, the neuron that those neurons synapse with, or contact and transmit their activity to. They're in the central system. But they're the ones contacted. So we talk about the cochlear nuclei, for example. They get input from the auditory nerve. The trigeminal nuclei in the hindbrain getting input from the skin of the face.
What's a motor neuron? Where is its cell body? Where's the cell body of a neuron innervating a muscle?
AUDIENCE: I was going to say it's right on top of the muscle.
PROFESSOR: No, it isn't. It's in the central nervous system. Any neuron in the central nervous system with a nerve fiber and axon leaving the central nervous system is a motor neuron. If it's an alpha motor neuron, it's contacting a striated muscle cell. So most of them are like that. But then there are also neurons like that that contact a cell in a ganglion. These are the autonomic nervous system cells. There you have a preganglonic motor neuron and then the ganglionic motor neuron.
What are all the other neurons? We have the primary sensory, secondary sensory, and motor neuron. What are those enormous numbers of neurons in between? The general term is they're interneurons. But in the field of neuroanatomy, usually the interneuron is a short axon cell. But really, all the long axon cells and the short axon neurons are all interneurons.
This is just a diagram that summarizes all those things I just told you. Some of you learn best if I describe it all verbally. Some of you have more trouble with that, but you see a picture like that and it's all obvious.
In the left screen, the color's a lot better, because I used green to describe some of those cells where I've got pointers going to them. So there's the primary sensory neuron. You see where the cell body is there? Everything within those two lines is the central nervous system, like the spinal cord. But it could be in the brain, too.
And there's the motor neuron with an axon going to a muscle cell. So I colored the motor neurons in. I've colored the primary sensory neuron. Secondary sensory neurons-- well in the book I'm writing I colored them green in the initial diagram, but I didn't color them green, here.
All right. So one of the major specialization of neurons that govern how we sense, how we move, how we think-- everything. How are those cells different from other cells? How are they different from liver cells and kidney cells, and skin cells, and so forth? Yes.
AUDIENCE: There are [INAUDIBLE] that transmit [INAUDIBLE]?
PROFESSOR: OK. That's a big one. That's a specialization of a neuron. They can transmit what we call action potentials over long distances down axons, because the axon is a specialized property of the neuron membrane-- of the axon. They have many processes that don't transmit action potentials, but they still respond to input.
So the general property here is this first one. We call it irritability. They respond to stimulation, and the response spreads. Even if it's not an action potential, you still get that spread.
Well, if you could record from cells in a leaf, and you might find potentials across the membrane, and it might respond to stimulation and spread. But they're rarely like action potentials.
So we call the response to a stimulus either mechanical or chemical that's being irritable or responsive to the outside world outside the cell. Conduction is that spread of potential where they have that extra specialization in the axon. It begins at a little place right near the cell body usually, near the beginning of the axon-- the axon hillock-- and the action that's where the action potential is initiated.
How is it different from the other potentials? Well, once it's triggered, it goes all the way down the axon. Unless something unusual happens to block it, it goes all the way down to the axon endings.
And there it can transmit its response to other cells at these contacts with other cells. We call those contacts synapses. Most of them are chemical synapses. And they cause a release of a chemical substance-- a neurotransmitter that affects the next cell. So these are the major specializations of nerve cells compared with any other cells in the body.
So let's talk about some of the things these nervous system cells do. We'll talk about an example used by Scott in his book. He talks about the wandering spider. It's a spider that doesn't make a web, but it still hunts insect prey.
How does it catch them without a web? It's got a lot of eyes, so you would think it's using its eyes. But you can blind them or block their eyes-- you can temporarily blind them just by covering their eyes with something-- and they still catch flies. How do they do it? Yes?
AUDIENCE: Well, they have a lot of hairs on their legs.
PROFESSOR: Little hairs, right?
AUDIENCE: Yeah. They can detect small motions [INAUDIBLE] disturbances.
PROFESSOR: Yeah, you're describing a pattern of disturbance in the air around a moving insect. Exactly. And this little spider has these little sensory hairs. Very interesting, because each hair, when it bends, there are at least four afferent sensory neurons-- they're called afferents-- sensory processes attached to that hair. So when the hair bends one way, one cell responds the most. It bends another way-- the opposite way-- another cell responds, and so forth. So in four different directions. And depending on its response, that one hair, you can tell basically where the direction of air current stimulating the hair.
And it seems to be born with the ability to use the pattern of responses in all those hairs covering its body. And there's lots of them-- over 100. And it can actually leap into the air and grab insects. Up to 20 centimeters away they can detect things. And it can leap several centimeters. So it can tell when the insect's approaching, when it gets close-- it sort of times its leap when it's close enough, and it can catch the insect. Very interesting. He's got a nice description. There's a box covering two pages, there. And they even have some neurophysiological results where they're recording from some of the neurons responsive to those hairs.
And of course, other kinds of spiders have other kinds of specializations. Usually they're responding to vibrations in the web when something is caught in their web. But these webless spiders have other means.
So let's talk a little bit about the toad. Frogs and toads have a very specialized system for catching prey. They also catch insects, like that spider. But here the specializations are in its visual system. There are cells in the retina that are specifically tuned to respond to small, usually darker-- the little stimulus in the visual field darker than the rest of the field, like a fly or another small insect. And these cells respond best when a fly-like stimulus is moving. So just a little spot will stimulate those cells, as long as it's moving.
Now those are in the retina. The retina's not enough to cause the frog to catch him. Where does the information go? Well, it goes down the optic nerve and to the opposite side of the roof of the midbrain, to the optic node of the midbrain. Or the optic tectum-- tectum just means "roof"-- the roof of the midbrain on the opposite side gets that input.
And what do we know about the way the retina maps, senses fibers to the midbrain tectum? Well, we know it forms an organized map. So one part of the retina projects the one part of that optic lobe. Another part of the retina projects to another part. So it forms a map to the retina in the brain. We have that in our midbrain, too-- as well as other parts of our visual system.
But in the frog, the dominant part by far-- and the only really important part for this prey catching-- is there in the midbrain.
So what else do frogs and toads eat besides flies? Don't you ever have pet frogs and toads?
AUDIENCE: Worms?
PROFESSOR: Worms. Right. They love worms as much as they love flies. What characterizes a worm? Well, it's got to be moving and longer than it is wide.
So if you make artificial stimuli and show them to the frog-- and this has been done by, say, placing the frog or the toad in the center of a turntable, and you put the stimuli on the platter, and you rotate it around, and you can put little black spots-- and you'll see that the frog keeps making these orienting movements towards it.
Well, if you put in the elongated worm-like object, he does the same thing. And if you make it longer and longer, he responds more and more.
What if you do the opposite? You make it wider and wider? He responds less and less. And if you start, say, with a square object, when it's little he does respond more and more. But then it reaches a certain size and it rapidly responds less and less. And it gets really big, and his whole response changes. He starts crouching. It starts being dangerous to him. These are all built-in responses-- innate responses-- of frogs and toads.
So we've already talked about where in the central nervous system of a toad-- and then we could say the same thing about frogs-- that input from the retina is going. So here I'm asking, where in the central nervous system of a toad could electrical stimulus elicit a prey-catching, fixed-action pattern?
Well, you could put an electrode in there. You anesthetize the frog, and implant the electrode. Let him wake up, recover from the surgery. Now just a little electrical stimulus in one part of that tectum-- that midbrain surface-- will elicit those turning movements that we were just talking about. So they can be elicited electrically.
The only problem is when you elicit them electrically, he never follows through. What does a normal frog do? He's following the worm. Then what does he do to get it? Flips his tongue out. His tongue comes out like a dart. And the tongue is sticky at the end. So, splat! He gets it, pulls it back.
Same for a fly. For the fly, he's tuned to wait until the fly alights. If it wasn't moving he will never try to get it. But if it was moving and stops, see the frog will just sit there and watch it move. But if it alights, then he'll get the prey-catching response-- if it's within range. He does have enough depth vision to know whether it's within range of his tongue.
When you stimulate it electrically, you never get the tongue flick. Why? Give me a reason. I can stimulate repeatedly. I can stimulate the turning movements. He keeps reorienting to different positions in the field every time I stimulate them. Why does he never flip his tongue? Isn't that all built-in? Isn't it part of the same fixed-action pattern?
Well normally, yes. Because normally, of course, just think. This is a frog without an electrode. He turns. That stimulus is moving across his tectum. He always brings it so the stimulus is in front of him. So he's aligned. So he can make the tongue flick response. Now it's always up in the front of the tectum. That's the part of the tectum which, if you stimulate, you can also get the tongue flick. But with just one electrode in the middle of the tectum, you'll never get it, because we can't imitate with a fixed electrode. We can't imitate the whole normal stimulus sequence.
Then here I say, what would change if an electrode were moved a short distance? Well, he would just change the position he's orienting towards. So you put it way in the back of the tectum, he would make these bigger orienting moves. If you put it closer to the front, he makes short orienting movements. If you place it closer to the midline, he orients upward. If you place it further out laterally, he orients to the side and downward.
Well, the map we have of the retina in our midbrain is the same as the map in the frog. It's conserved across all the vertebrates. The relative size of structures changes a lot, but the existence of these structures is the same throughout the vertebrates. And that's true for all the structures of the spinal cord and brain stem that control these fixed-action patterns.
Another concept here. We talked about what a motor neuron is. If the motor neuron's stimulated, you get a muscle twitch. If a group of motor neurons are stimulated, you can get more movement. And if I stimulate all the motor neurons in the cord, I can get suddenly rigidity if I'm stimulating, say, in the motor neurons controlling my hand. But if I'm stimulating just one group of them I can get flexion of the hand. I stimulate another group, I can get-- this would be extension, this would be flexion. So I could elicit grasping.
What about a command neuron? It has a different meaning, also very important in studying the control of these movements in fixed-action patterns. What is a command neuron? It's a term used in neuroethology to describe a neuron that you can identify. Usually it's a neuron. You can identify the same neuron in one member of the species to another. Sometimes they even have different color pigments.
It's a neuron where if you stimulate that one neuron you stimulate a whole pattern of movement. You get a behavior from one neuron. You stimulate a command neuron in a crayfish, you can elicit, if you're in the right place, you stimulate the right command neuron, you can get a tail-flip response. The animal will escape as if escaping from a predator.
Mammals and vertebrates in general-- it's harder to find the command neuron. There are a few very large neurons that you can identify, say, across fish and amphibians. There's very giant cells in the hindbrain they call the Mauthner cells that can elicit rapid escape responses. They're like command neurons. But otherwise, usually these are insects and other invertebrates.
All right. That's the difference between a command neuron and a motor neuron. In a vertebrate you have groups of neurons though that act as command groups of neurons, which when stimulated can elicit a whole sequence of movements-- for example, in the caudal part of the midbrain. Below the optic tectum-- below in fact the auditory tectum-- it's in the caudal midbrain-- there's an area there which, when activated, elicits locomotion. You get the right place and you stimulate with enough activation, you can get rapid running movements.
There are similar groups of neurons further forward in an area we call the subthalamus. Parts of the subthalamus get direct visual input from the retina. And more immediately, they can activate these neurons that seem to connect directly to that midbrain center, because they also elicit rapid running movements usually involved in escape behavior. That's a kind of command neuron group. It's just that we can't identify the single neuron. So if I say "command neuron," I'm almost always talking about invertebrate animals where we can actually identify the same cell from animal to animal.
So let's talk about the giant axon. What is the main advantage of the giant axon in the triggering of an escape response? You see giant axons in squid. It was the discovery of giant axons in squid that led to the detailed physiology of the action potential for the first time. Because this was before microelectrodes were perfected. They could get a tiny wire inside that giant axon. They were up to one millimeter in diameter in a squid. So it enabled detailed study of the physiology of the action potential in the giant axon.
But why would the squid have giant axons? They use a lot of energy. If all their axons were like that, they'd be gigantic animals. Some of them are pretty large, but they're not gigantic. And some of them are small, too, but all of them have these giant axons. Why? What's the advantage of making an axon larger in diameter?
AUDIENCE: They have more rapid responses?
PROFESSOR: Yeah, one word-- speed. Rapid conduction. That's why giant axons evolved. Because axonal conduction speed depends first of all on the diameter of the axon. So you get faster conduction of the action potential. Well, we have rapid conduction too, and we don't have giant axons. We do have some axons larger than others, and they do conduct more rapidly, but they're nowhere near the size of the squid. And yet we get conduction that's just as fast as in the squid-- probably faster. I can't remember the exact speed of conduction in a giant axon, but I know the long axons down to our spinal cord are very rapidly conducting.
The reason is the evolution of the myelin sheath. Myelin is when the axon is wrapped round and round by the membrane of a glial cell that sort of insulates the axon from the ionic potentials that normally are involved in the action potential. Well, if you insulated the whole axon like that, you wouldn't get any action potentials at all. So this is why it gets-- first of all, deal with the rate of spread of current down an axon.
For all you engineers, this should make sense to you. If you're not engineers, well, be patient. You've studied basic physics and math. The rate of passive spread of current down the center of an axon is proportional to the reciprocal of this product. The resistance of the axon down the cylinder can be measured, and the capacitance of the membrane.
You can increase the capacitance with myelin. You can decrease the resistance simply by making the axon bigger around. I point out down here that the resistance decreases in proportion to the square of the diameter of the axon, and the capacitance increases in proportion to the diameter.
So then how does the action potential move? We know that the myelin makes that passive conduction the passive spread of current-- the kind of spread you get in the cell body where you touch the cell in one place-- you stimulate it in one place-- it does spread to the rest of the cell. But not if it's not an action potential. In fact, it gets detrimental. It gets weaker the further you get from where you stimulate. The same is true in all those little processes of the cell we called dendrites. It spreads passively.
Here's a greatly enlarged axon-- this long cylinder. That's the axon membrane. The way the myelin forms is-- let's say this is a peripheral axon in our leg. We'll draw the cell body forming that. Here's the nucleus. That cell, its membrane is wrapped round and round and around this portion of the axon. This is a cross-section.
And then there's another cell wrapping its membrane here. Another one here wrapping its membrane. These cells are called Schwann cells. They're in the periphery.
If this was in the central nervous system, in the brain, say, where we also have a lot of myelin, same in the spinal cord-- then there would be a cell up here forming myelin, and like this. And it would have these branches spreading down and wrapping the membrane round and round and around the axon. So the same cell here can myelinate a whole bunch of axons. That's an [INAUDIBLE] site in the brain.
So the same thing is accomplished. These cells are insulating the axon. So how does that help? If the action potential, say, is triggered here, we know that this depolarization of the membrane spreads very fast down the axon. No action potential until you get to the next node here, where there's no myelin. It triggers an action potential there.
Then that spreads decrementally down to the next node. The spread is extremely rapid with a large diameter and with the insulated membrane. It keeps triggering the action potential in node after node after node-- that's called saltatory conduction-- jumping conduction. And it just goes b-r-r-a-p! And it goes very, very fast without making the axon so gigantic.
So that's how it happens. And that's the end of the class. And we'll talk about motivational states next time.